Subscribe to RSS
DOI: 10.1055/a-2186-3557
Mechanisms of Lipid Droplet Accumulation in Steatotic Liver Diseases
- Hepatocyte LD Biology
- Metabolic Dysfunction-Associated Steatotic Liver Disease (Formerly, Nonalcoholic Fatty Liver Disease)
- Alcohol-Associated Liver Disease
- Hepatitis C
- Survey of Techniques to Study LDs in Steatosis
- Leveraging LD Biology for Therapeutics
- Conclusion
- References
Abstract
The steatotic diseases of metabolic dysfunction-associated steatotic liver disease (MASLD), alcohol-associated liver disease (ALD), and chronic hepatitis C (HCV) account for the majority of liver disease prevalence, morbidity, and mortality worldwide. While these diseases have distinct pathogenic and clinical features, dysregulated lipid droplet (LD) organelle biology represents a convergence of pathogenesis in all three. With increasing understanding of hepatocyte LD biology, we now understand the roles of LD proteins involved in these diseases but also how genetics modulate LD biology to either exacerbate or protect against the phenotypes associated with steatotic liver diseases. Here, we review the history of the LD organelle and its biogenesis and catabolism. We also review how this organelle is critical not only for the steatotic phenotype of liver diseases but also for their advanced phenotypes. Finally, we summarize the latest attempts and challenges of leveraging LD biology for therapeutic gain in steatotic diseases. In conclusion, the study of dysregulated LD biology may lead to novel therapeutics for the prevention of disease progression in the highly prevalent steatotic liver diseases of MASLD, ALD, and HCV.
#
Keywords
steatotic liver disease - lipid droplet - lipid droplet proteins - perilipins - MASLD - alcohol-associated liver disease - hepatitis CLipid droplets (LDs) are a metabolically active component inside of cells. In the liver, LDs are made from fatty acids and are used to store other lipids, lipid toxic compounds, and signaling molecules. The fats stored inside an LD or at the surface can be used for the cell membrane or for energy. However, two diseases—metabolic dysfunction-associated steatotic liver disease (MASLD) and alcohol-associated liver disease (ALD)—are caused by the overaccumulation of LDs, and hepatitis C uses LDs for its viral life cycle. By understanding the development of LDs in three of the most common liver diseases, we may be able to develop novel therapeutics to prevent disease progression.
Lipid droplet (LD) organelles are highly conserved organelles that are at least 3.1 billion years old.[1] LDs are the intracellular hubs of lipid metabolism in all cells and are increasingly acknowledged as the disease-defining organelles of several hepatic steatotic diseases. Debated as a bona fide organelle as recently as 2006,[2] LDs have since emerged as the organelles that harbor most of the genetic risk for two common hepatic steatotic diseases, metabolic dysfunction-associated steatotic liver disease (MASLD, formerly called nonalcoholic fatty liver disease) and alcohol-associated liver disease (ALD). LDs are also required for the viral life cycle of the third major hepatic steatotic disease, hepatitis C virus (HCV). Thus, for three of the most common causes of liver disease worldwide, LD organelles are intimately involved in disease susceptibility and pathogenesis.
The advent of advanced imaging and molecular and genetic tools has both increased our understanding of the biology of LDs and prompted new, yet still unexplored questions about this organelle. For example, much is now known about the biogenesis of LDs and the possible fates of LDs. Less is known about the signals that regulate LD size and composition and how the potential fates are determined. In addition, while our understanding of LD biology and consequent pathogenesis in some diseases like MASLD appears to have garnered consensus,[3] our understanding of other diseases like ALD and HCV lags.
Here, we review the current understanding of hepatocellular LD biology, from their formation as nascent LDs to their involvement in the pathogenesis of three diseases, MASLD, ALD, and HCV. We also take this opportunity to outline gaps in our current understanding as they relate to these diseases and highlight recent efforts to leverage LD biology for therapeutics in steatotic diseases.
Hepatocyte LD Biology
The nascent LD emanates from the outer membrane of the endoplasmic reticulum (ER; [Fig. 1]). This process is mainly driven by the coordinated activity of enzymes such as diacylglycerol O-acyltransferase 1 (DGAT1) and DGAT2 and acetyl-CoA acetyltransferase 1 (ACAT1) and ACAT2, which esterify fatty acids (FAs) and cholesterol, respectively. DGAT1 is only present in the ER membrane and uses diacylglycerol (DAG) derived from cytosolic triglyceride lipolysis to re-synthesize triglycerides in the ER lumen. DGAT2 localizes to both the ER and the LD membrane and synthesizes de novo triglycerides from FAs.[4] [5] ACAT1 converts cholesterol into cholesteryl esters in the cytosol which are then incorporated into LDs to prevent cytotoxicity from cholesterol crystals. However, the product formed by ACAT2 is translocated to the ER and released in lipoproteins.[6] [7] The resulting neutral lipids, namely triglycerides and cholesterol esters, form the core of hepatocellular LDs. The budding of nascent LDs from the ER outer membrane is facilitated by phase separation of neutral lipids within the ER bilayer, the direction of LD curvature (i.e., positive curvature toward the cytosol or negative curvature toward the ER lumen), and the engagement of several proteins that comprise the LD assembly protein complex.[8] [9] [10] [11] Here, the protein seipin plays a critical role. Seipin is an evolutionarily conserved protein required for LD formation and budding. Recently, a model was proposed that seipin forms a cage-like structure whose conformation determines either triglyceride phase separation or LD growth and budding.[12] Seipin and other proteins involved in the LD assembly protein complex ultimately determine initial LD size and composition.[13] [14] [15] [16] [17] The mature hepatocyte LD is composed primarily of a neutral core of triglycerides and cholesterol esters. We have also demonstrated that the LD fraction includes bioactive lipids such as ceramides whose accumulation interferes with insulin signaling and other essential cellular processes.[18] [19]


The neutral lipid core is enveloped by a phospholipid monolayer of multiple LD proteins. These proteins can either traffic from the ER bilayer to the LD membrane (Class I LD proteins) or remain associated with cytosolic LDs (Class II LD proteins). Patatin-like phospholipase domain-containing protein 3 (PNPLA3), a Class I protein, is particularly noteworthy, as it increases the risk of MASLD and ALD.[20] [21] [22] [23] [24] In hepatocyte LDs, perilipin (PLIN) proteins predominate as Class II proteins. There are five types of PLINs (PLINs 1–5), and others and we have demonstrated that in addition to regulating lipid metabolism, these proteins are involved in glucose metabolism.[25] [26] [27] Another Class II protein family is the CIDE family, a polymorphism of which has been associated with reduced risk of MASLD.[28] [29]
Once formed, the mature LD has several potential fates largely dependent on the cell's nutrient state. The LD can remain a storage vesicle for neutral lipids; be catabolized through lipolysis or lipophagy whose release of FAs can be used for FA oxidation; or undergo repackaging into very low-density lipoprotein (VLDL) for secretion. An imbalance between LD formation and catabolism leads to hepatic steatosis. Steatosis, in turn, can cause the signature molecular, structural, and functional changes that characterize the initial stages of MASLD, ALD, and HCV.
#
Metabolic Dysfunction-Associated Steatotic Liver Disease (Formerly, Nonalcoholic Fatty Liver Disease)
MASLD is a chronic liver condition characterized by the accumulation of LDs in more than 5% of liver mass.[30] [31] MASLD is a progressive condition that can advance to steatohepatitis (MASH [metabolic dysfunction-associated steatohepatitis])—defined as hepatocyte injury due to inflammation and hepatocellular ballooning. Steatosis in MASLD is categorized as macrosteatosis (hepatocytes with large LDs and a peripherally displaced nucleus) or microsteatosis (hepatocytes with small [typically less than 1 micron] LDs in a foamy appearing cytoplasm with a centrally positioned nucleus), the latter phenotype portending a worse prognosis ([Fig. 2]).[32] The progression of steatosis to MASH can be followed by the development of fibrosis, liver cirrhosis, liver failure, and potentially hepatocellular carcinoma (HCC).[33] [34] While the mechanisms behind the pathogenesis and progression of MASLD are not fully elucidated, LDs play a critical role.


In the context of MASLD, LDs serve major functions. First, LDs are sites of lipid synthesis and storage to protect the cell from cytotoxic FAs and other bioactive lipids. Second, through neutral lipid lipolysis or autophagy, LDs are also important sources of the FAs used to synthesize essential lipids needed for membrane biogenesis. Although dysregulation of this balance leads to an accumulation of LDs, the increased LD capacity remains insufficient to mitigate the ER-stress-inducing FAs. And in some individuals who harbor genetic polymorphisms of LD proteins, the inability to depend on counterregulatory mechanisms that protect against LD accumulation adds insult to injury.[34] Ultimately, this unmitigated LD accumulation has effects on both hepatocyte and microvasculature structure which spurs progression to MASH.
LDs and ER Stress in MASLD
A reduced capacity of LDs to successfully bud from the ER bilayer membrane can lead to lipotoxic ER stress, a widely acknowledged catalyst for MASLD progression.[35] LD budding from the ER is normally a highly regulated process that depends on the aforementioned phase separation of neutral triglycerides, the directionality of LD curvature, and the engagement of LD proteins. In MASLD, hepatocytes are exposed to excess FAs which are then directed to the ER for esterification. The accumulation of bioactive FAs such as DAGs favors negatively curved nascent LDs that fail to bud from the ER membrane and consequently have reduced access to the LD proteins that promote neutral lipid LD storage.[36] The disruption in ER homeostasis is called ER stress and has implications for other normal ER functions, including its regulation of the unfolded protein response (UPR). Dysregulation of the UPR leads to an accumulation of unfolded or misfolded proteins in the ER, and the resultant proteotoxic ER stress can lead to lipid dysregulation and the further accumulation of lipids in the ER.[37] An unmitigated UPR can also lead to hepatic inflammation, inflammasome activation, and cell death.[38]
#
Genetics of the LD Proteome in MASLD
The mature LD engages multiple LD proteins and enzymes involved in lipid metabolism, membrane trafficking, and protein degradation. The five PLIN LD proteins predominate in hepatocytes, and several PLINs have known roles in MASLD. In general, PLINs promote LD structure and maintain the integrity of the neutral lipid core. While PLIN1 is predominantly expressed in adipocyte LDs, it can be de novo expressed in both adult and pediatric nonalcoholic steatohepatitis (NASH).[39] [40] [41] The exact role of this upregulation in MASH is unknown but may relate to the established role of PLIN1 in inhibiting lipolysis—a function that would serve to reduce the burden of FAs.
PLIN2 is the most abundant perilipin LD protein and, others and we have demonstrated its upregulation in MASLD.[39] [41] PLIN2 promotes storage of neutral lipids within large LDs by impeding access of the lipolytic enzyme adipose triglyceride lipase (ATGL); inhibiting FA oxidation; and protecting against lipophagy.[42] We have also demonstrated that PLIN2 promotes insulin resistance through the positive regulation of ceramides—another potential mechanism for lipotoxicity and oxidative stress in MASH.[26] [43] [44] [45] Recently, the PLIN2 S251P polymorphism was discovered to promote the accumulation of small LDs[46] and increase insulin sensitivity.[47] While there may be an increased association of the polymorphism with MASLD,[46] [48] we have not found an increased prevalence in patients with MASLD when using a large and diverse population dataset (published abstract).[49]
Less is known about PLINs 3–5 in MASLD. PLIN3 is a ubiquitous LD protein expressed on nascent and small LDs. In the liver, PLIN3 (like PLIN2) is found in both hepatocytes and hepatic stellate cells in humans with MASLD.[39] In mice, PLIN3 promotes steatosis and insulin resistance, and conversely, silencing of PLIN3 reduces steatosis and improves insulin sensitivity.[25] PLIN4 is highly expressed in adipose tissue,[50] but to date, there have been no reports of its role in MASLD. PLIN5 is expressed in oxidative tissues, hence its prior name of Oxpatperilipin (OXPAT). These tissues include brown adipose tissue, cardiac and skeletal muscle, and liver.[51] [52] PLIN5 regulates hepatic triglyceride metabolism through promoting LD storage and inhibiting lipolysis.[52] [53] PLIN5 expression is increased in MASLD[54]; however, unlike PLIN2, PLIN5 tends to protect against hepatic insulin resistance providing evidence that steatosis per se does not impair hepatic insulin signaling.[55]
While there is still much to learn about the role of perilipin proteins in the context of MASLD, these studies do demonstrate the critical roles of the LD proteome. Indeed, we recently estimated that there are at least 77 genetic polymorphisms involved in MASLD pathogenesis, and many of these genetic variations are in LD protein genes.[56] The most validated of these proteins is the Class I ER bilayer LD protein PNPLA3. The substitution of isoleucine to methionine in position 148 (I148M) of PNPLA3 causes a missense variant PNPLA3 I148M that fails to disengage from LDs due to a defect in its ability to be degraded by the ubiquitin proteosome system or by autophagy.[20] [24] Because this variant PNPLA3 also demonstrates reduced hydrolase activity, LDs accumulate in individuals who harbor this polymorphism. The consequent increased MASLD risk and severity associated with this variant[57] occurs in the absence of effects on insulin sensitivity.[58] Exactly how the polymorphism promotes liver disease progression is unknown but may result from the variant-induced shifts of the LD lipid composition toward higher polyunsaturated FAs and reduced phosphatidylcholine.[59] Reduced phosphatidylcholine impairs the hepatocyte's ability to synthesize VLDL particles[60] (which further compromises the cell's ability to reduce the burden of hepatocyte lipids) and increases membrane fluidity, the consequence of which can be loss of cell membrane integrity.[61]
While several genetic polymorphisms of LD proteins tend to increase MASLD risk and promote its pathogenesis, others are protective. For example, a polymorphism of the LD protein hydroxysteroid 17-β dehydrogenase 13 (HSD17B13) results in a splice variant (rs72613567:TA) that codes for a truncated protein. This variant is not associated with changes in LD neutral lipid accumulation but is associated with reduced aminotransferase levels, higher platelet counts, and protection against the progression of steatosis to NASH.[62] A loss-of-function variant (rs143404524) was subsequently reported in black and Hispanic individuals that had no effect on steatosis. These data suggest that the benefit of the HSD17B13 polymorphism stems not from its global effects on LD concentration but rather from its effects on LD lipid composition. Indeed, the HSD17B13 splice variant increases several hepatic phospholipids, including phosphatidylcholine.[63] In contrast to the expected effects of the PNPLA3 variant on membrane fluidity, this shift is likely to stabilize membrane fluidity and integrity, thus improving overall cell function. Notably, in individuals who have both the HSD17B13 splice variant and PNPLA3 I148M polymorphism, HSD17B13 mitigates the effects of the PNPLA3 polymorphism demonstrating that the interplay of LD proteins is as critical to understand as their individual functions.
The most recent LD protein genetic polymorphism to have gained attention in its ability to reduce MASLD risk is cell death-inducing DNA fragmentation factor α-like effector B (CIDEB).[28] CIDEB is a Class I LD protein that is highly expressed in hepatocellular LDs, the ER membrane, and Golgi apparatus.[64] It has several known functions including promoting LD–LD lipid transfer, fusion of small and large LDs, and lipidation and maturation of VLDL particles within the Golgi apparatus.[65] [66] Loss of function and missense CIDEB variants are associated with lower liver fat and aminotransferase levels and protect against MASLD, cirrhosis, viral hepatitis, and ALD, thus demonstrating CIDEB's (and by extension, LD proteins') broad role in normal hepatic function.[28] The protection garnered by these CIDEB polymorphisms is most pronounced in obese individuals and in those with PNPLA3 polymorphisms, making CIDEB modulation an attractive therapeutic target for individuals with the highest MASLD risk.
#
LD Effects on Hepatic Morphology in MASH
The idea of steatosis as a benign or “simple” stage of disease has not been borne out by what we now understand about MASLD pathogenesis. Indeed, there are a myriad of molecular and genetic reasons why LD accumulation is pathologic to the liver.[34] Emerging data also demonstrate that LD accumulation has deleterious effects on both hepatocellular and hepatic structures that spur NASH development.
MASH is classically defined as the presence of ballooned hepatocytes, which can be found on routine hematoxylin and eosin (H and E) staining, but the clinical reliability and utility of this designation has come under scrutiny.[67] Ballooned hepatocytes are generally thought to be a step beyond steatosis and are characterized by substantial LD accumulation, dilated ER, and cytoskeletal injury with Mallory–Denk body formation.[68] [69] [70] These large, deranged hepatocytes represent a lipotoxic phase of injury in which FAs and free cholesterol are released from the LD core, thus causing hepatic inflammation.[71] Lipotoxicity is exacerbated by the activation of c-Jun M-terminal kinase signaling pathways that cause cellular stress, inflammation, apoptosis, mitochondrial dysfunction, and inhibit B-oxidation.[72] [73] [74] While much attention has been paid to the MASH-defining ballooned hepatocyte, less well recognized are the ultrastructural changes that can occur because of hepatocyte LD accumulation during steatosis.
Steatosis itself impairs both hepatic blood flow and the hepatic parenchymal microcirculation. In humans with MASLD, sinusoidal portal hypertension is related to steatosis grade.[75] In vitro, large LDs can also distort hepatocyte nuclei and chromatin structure.[76] In preclinical rodent models, LD-laden hepatocytes compress the space of Disse, the subendothelial space that lies between the liver sinusoidal endothelial cells and hepatocytes. Under normal conditions, this region regulates blood flow (70% portal vein and 30% hepatic artery).[77] In severe MASLD, compression of the space of Disse by the steatotic hepatocytes causes hypoxia by increasing intrahepatic resistance and portal venous pressure, thereby reducing oxygen supply. Tissue hypoxia stimulates angiogenesis and type I collagen,[78] promotes inflammatory cytokines, and activates hepatic stellate cells that results in extracellular matrix deposition and fibrosis development.[57] In rabbits given high cholesterol diets, sinusoidal blood flow was reduced by half due to moderate steatosis and was decreased further in rabbits with severe steatosis.[79] In rats fed a methionine–choline-deficient diet for several weeks, significant portal hypertension was observed in severely steatotic rats despite the absence of significant inflammation or fibrosis on standard H and E.[80] In scanning electron microscopy, mouse steatotic hepatocytes are observed to directly constrict and distort sinusoids. Capillarization (i.e., defenestration) of sinusoids ensues which impairs hepatic perfusion, promotes space of Disse collagen deposition, and traps leukocytes, which then incite a NASH-inducing inflammatory cascade.[81] These ultrastructural changes that result from hepatocellular LD accumulation cannot be detected on routine H and E and do not initially lead to overt clinical manifestations. This may explain why steatosis has often been considered a benign, nonpathologic stage of liver disease. To the contrary, hepatocellular LD accumulation can lead to significant remodeling of the hepatic microenvironment which over time promotes the progression from steatosis to advanced stages of MASLD.[82]
Clinically, steatosis in the context of MASLD is an independent predictor of patient outcomes and is associated with the onset of other metabolic diseases. Steatosis progressively increases the risk of type 2 diabetes. There is up to a four times risk of incident risk of diabetes development after steatosis develops and this risk increases up to 15-fold as steatosis severity worsens. Conversely, steatosis resolution can reduce the risk of incident diabetes on follow-up to a level comparable to individuals who have never had steatosis.[83] Steatosis also increases the risk of cardiovascular disease. Compared with patients who do not have steatosis, those with steatosis have a hazard ratio of 1.69 for cardiovascular events. Moreover, this risk is independent of other cardiovascular risk factors or amount of cardiac arterial stenosis.[84] There is also an association between hypertension and steatosis,[85] and steatosis is a predictor of mortality in acute myocardial infarction with hepatic fibrosis having only a slightly increased risk of mortality compared with steatosis.[86] Finally, steatosis more than 30% predicts graft failure when steatotic livers are transplanted.[87] While the specific mechanisms of this graft failure have not been fully established, it is likely that the aforementioned effects of LD accumulation on the hepatic microenvironment and tissue oxygenation impact graft suitability. These studies demonstrate that steatosis per se matters and that MASLD is not benign. To the contrary, steatosis is predictive of and associated with chronic metabolic diseases and death.
#
#
Alcohol-Associated Liver Disease
ALD is a major cause of liver disease worldwide and is responsible for over 50% of cirrhosis-related deaths.[88] [89] Hepatic steatosis is the initial stage of ALD and results from alcohol's profound, deleterious effects on hepatic lipid metabolism and LD biology.[90] [91]
Mechanisms of LD Accumulation in ALD
Although not as prominent as in patients with MASLD, increased de novo lipogenesis plays a role in ALD pathogenesis ([Fig. 3]). Through the increased activation of several transcription factors, including sterol regulatory element binding transcription factor 1 (SREBF1/SREBP1c),[92] [93] [94] [95] [96] [97] [98] MLX interacting protein like (MLXIPL/CHREBP),[99] [100] and peroxisome proliferator activated receptor gamma (PPARG),[101] alcohol increases hepatic FA synthesis. In addition to alcohol's promotion of FA synthesis, chronic alcohol increases hepatic uptake of nonesterified FAs. Through both exaggerated adipose tissue lipolysis[102] [103] and increased hepatic clearance of intestinal chylomicrons,[104] [105] alcohol causes an influx of nonesterified FAs. This influx can be abrogated by genetic deletions of key enzymes such as abhydrolase domain containing 5 and lysophosphatidic acid acyltransferase (ABHD5), an enzyme involved in adipose tissue lipolysis.[106] Alcohol also causes increased hepatic uptake of FAs through the upregulation of hepatic FA transporters. Namely, alcohol increases hepatic FA translocase/CD36, which normally has low hepatic expression.[107] As with ABHD5 inhibition, CD36 ablation prevents alcohol-induced hepatic lipid accumulation.[108] [109] Finally, FAs can accumulate in the liver through impaired mitochondrial β-oxidation. Here, alcohol has two major effects on β-oxidation: excessive alcohol consumption damages mitochondria[110] [111] [112] [113] and alcohol interferes with FA oxidation in favor of alcohol oxidation.[114] [115]
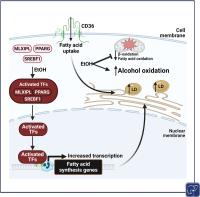

Compounding the alcohol-induced increase in hepatic FAs is the inhibition of FA export and lipophagy. Normally, neutral lipids are exported from cells after re-incorporation into VLDL particles. VLDL is synthesized in the ER after lipidation by apoB100 and matures in the Golgi apparatus. MTTP is a key enzyme that regulates VLDL synthesis, and alcohol impairs both MTTP and apoB100 syntheses. Alcohol also reduces the levels of S-adenosyl methionine (SAM) and the SAM-dependent enzyme phosphatidylethanolamine methyltransferase, which is responsible for the production of the phosphatidylcholine component of VLDL.[116] [117] [118]
The increase in intracellular lipids can be exacerbated by the inhibition of lipophagy by chronic ethanol exposure. Rab7 (member of the RAS oncogene family)—a small guanosine triphosphatase that regulates membrane trafficking—mediates hepatocellular lipophagy. Rats fed an alcohol diet were resistant to starvation-induced lipophagy likely through inhibition of Rab7; rat primary hepatocytes exposed to ethanol had similar characteristics.[119] Alcohol also has a direct effect on the biogenesis and recycling of lysosomes that actively remove LDs. Transcription factor EB (TFEB), which can promote microlipophagy and macrolipophagy, mediates lysosomal biogenesis for the removal of LDs. In mice, alcohol decreased TFEB-mediated lysosome biogenesis, whereas overexpression of TFEB protected mice from alcohol-induced steatosis.[120] [121] In rats, activity of dynamin 2 (Dnm2/Dyn2), which is responsible for recycling lysosomes to maintain autophagy, was inhibited by alcohol and decreased lysosomes by 40%.[122] The mechanisms by which ethanol regulates Rab7, TFEB, and Dyn2 are unknown. Notably, we have demonstrated in an experimental model of ALD that restoration of lipophagy can be achieved by hepatic ceramide reduction,[18] demonstrating that the effects of alcohol on lipid pathways may be reversible. Characterizing the mechanism of ALD abrogation by ceramides may provide targets for inducing lipophagy and alleviating alcohol-induced steatosis.
#
Alcohol and LD Proteins
Given the alcohol-induced increase in FA uptake and synthesis, it is not surprising that there is an attempt by hepatocytes to upregulate pathways that promote esterification and safe neutral lipid storage as a strategy to reduce lipotoxicity. For example, in mice, alcohol increases LD size through increased expression of the LD protein cell death inducing DFFA like effector C (Cidec/Fsp27),[123] which in humans is correlated with the degree of steatosis in patients with ALD.[124]
Alcohol also upregulates the LD protein PLIN2 whose role is to prioritize triglyceride storage at the cost of worsening LD catabolic processes such as lipolysis and lipophagy. Indeed, we have demonstrated experimentally that PLIN2 is required for the development of alcoholic steatosis and alcohol-induced insulin resistance.[26] [43] However, we also observed that PLIN2-enveloped LDs harbor bioactive ceramides whose accumulation impairs insulin signaling. Thus, there appears to be an exquisite balance between healthy lipid storage within LDs and LD stagnation wherein the LD-associated lipids have no opportunity for catabolism. Catabolism of LDs is aided by LD mobility so that LDs can interact with other organelles. Here, PLIN3 attempts to exert a protective effect against intrahepatocellular accumulation of LDs. PLIN3 is an exchangeable and cytosolic perilipin protein whose expression is induced in mice chronically exposed to ethanol. In basal conditions, PLIN3 surrounds small, nascent LDs and spatially organizes LDs through the co-localization of the microtubule dynein subunit Dync1i1. While alcohol can upregulate PLIN3, alcohol disrupts the LD–microtubule interaction which results in small LDs being dispersed throughout the cytoplasm,[125] so-named microsteatosis. Alcohol also induces microtubule acetylation, which further impairs LD motility.[126] Thus, the overall effect of chronic alcohol exposure on the liver is unmitigated LD accumulation with little opportunity for LD catabolism. Akin to MASLD, this can lead to ER stress, inflammation, and hepatocyte cell death.
#
Genetics of the LD Proteome in ALD
Because of alcohol's myriad effects on lipid metabolism, it is not surprising that the majority of the known ALD genetic risk is attributable to LD proteins.[127] [128] For example, the PNPLA3 missense I148M mutation is the highest risk allele for the development and severity of ALD.[20] [129] The MBOAT7 rs641738 C > T variant is associated with an increased risk of steatosis and fibrosis, although the exact mechanisms are unknown.[130] In addition, as in MASLD, HSD17B13 variants reduce the risk of cirrhosis and HCC in chronic alcohol users. Using four European cohorts, investigators examined 6,171 patients who either had alcohol-associated cirrhosis with or without HCC, were chronic alcohol misusers without liver disease, or were normal, healthy controls without a history of alcohol misuse or liver disease. The HSD17B13 variant reduced the risk of cirrhosis by approximately 15% and, in men, reduced the risk of HCC by 10%.[131] Taken together, increased alcohol-induced hepatic FA uptake, inhibition of lipid export, reduction of FA oxidation, and promotion of de novo lipid synthesis and LD biogenesis are all pathways that converge with underlying genetic LD risk factors to determine one's individual susceptibility to ALD.
#
#
Hepatitis C
Hepatitis C is a chronic liver condition caused by the HCV that can cause hepatic steatosis, chronic hepatic inflammation, fibrosis, cirrhosis, and HCC. In the early 2000s, HCV was estimated to affect approximately 170 to 185 million people, but effective treatments have brought the global prevalence of this disease to approximately 58 million in 2019.[132] [133] [134] While the effects of HCV on liver inflammation are quite well understood, the effects on liver metabolism are increasingly being uncovered aided in part by the observation that HCV genotypes have differential effects on lipid metabolism. Namely, patients with HCV genotypes 1, 2, and 3 are prone to steatosis, but the etiology of steatosis in genotype 1 and 2 patients appears to stem from obesity, insulin resistance, and metabolic syndrome while steatosis in genotype 3 occurs in normal weight individuals.[135] While these differences may be confounded by differences in viral geographic distribution (and hence regional differences in body mass indices), there does appear to be true biologic differences in how these viruses negotiate hepatic lipid metabolism as well.
Lifecycle of HCV
HCV is a single-stranded, positive-sense RNA virus that relies on interactions between viral and host proteins for replication. First, the HCV virion circulates in the bloodstream as a low-density lipoprotein-viral particle (lipo-viral particle) or as a free particle ([Fig. 4]).[136] The virion enters the cell via endocytosis through interactions with cell-surface receptors. The positive-strand RNA genome is released into the cytoplasm where it undergoes ER translation. Ten distinct proteins derive from cleavage of the polyprotein precursor by host and viral proteases.[137] Viral replication is accomplished through the engagement of a viral complex formed by both nonstructural viral and host proteases. Core is the first translated protein and forms the capsid which interacts directly with cytoplasmic host LDs.[138] [139] [140] The virion envelope is formed by the ER-interacting transmembrane E1 and E2 glycoproteins. Replicated viral RNA is trafficked to the virion by nonstructural protein 5A (NS5A), wherein the nucleocapsid is formed around the HCV genome.[141] [142] [143] Ultimately, infectious lipoviral particles are formed from the association of the mature virion with endogenous lipoproteins sourced from the core–LD interactions.[144] In addition to E1 and E2, HCV uses the host VLDL assembly mechanisms, such as apolipoprotein B (ApoB)[145] and ApoE,[146] to coat the nucleocapsid. The coating of the nucleocapsid by host proteins allows the virus to easily exit an infected cell and enter a new target cell.


#
HCV-Induced Steatosis
Steatosis is a hallmark of HCV infection and results from HCV protein interactions with the LD or lipid metabolic pathways.[147] The structural core protein increases the number of LDs and shifts the size distribution of LDs toward smaller droplets.[148] LD lipid composition appears to depend on HCV genotype, as core protein in HCV genotype 3a increases LD cholesteryl ester content and liver ceramides,[149] bioactive lipids that we have shown impair insulin signaling.[19] Core co-localizes with the ER membrane-associated enzyme DGAT1, which is required for efficient HCV assembly. With increased expression of the core and the overexpression of DGAT1, these two proteins gradually co-localize to the LD.[150] DGAT1 is also responsible for LD localization of NS5A from the ER. NS5A facilitates the translocation of other HCV nonstructural proteins, and inhibition of NS5A prevents the translocation of other nonstructural proteins, indicating a stepwise transition for HCV interaction from the ER to the LD.[151] Additionally, DGAT2, which is localized to the LD and is responsible for LD expansion,[152] does not associate with core or NS5A, reinforcing that DGAT1 must be functionally present for the HCV replication cycle.[150] [153]
HCV also has an impact on lipogenesis. In mice, HCV activates SREBP1c to induce de novo triglyceride synthesis while also inhibiting VLDL secretion of triglycerides and promoting LD formation.[154] Also, HCV induces lipogenesis by the downregulation of HNF4A. HNF4A regulates the expression of the microRNA mir-122, thereby inhibiting NF-κB-inducing kinase (NIK), an upstream lipogenic regulator of IκB kinase α (IKK-α). Decreased expression of HNF4A decreases mir-122 expression and leads to activation of NIK and IKK-α, promoting lipogenesis.[155] Conversely, HCV infection in liver cells can inhibit de novo lipogenesis by decreasing the expression of the long noncoding RNA Linc-Pint. Linc-Pint binds to serine/arginine-rich protein-specific kinase 2, which induces efficient splicing of genes in the lipogenesis pathway such as FA synthase.[156] Because HCV is known to induce steatosis, the decreased expression of Linc-Pint and subsequent inhibition of lipogenesis suggests that modulation of lipid metabolism by HCV is stage- and pathway-dependent. Additionally, HCV assembly uses α/β hydrolase domain-containing protein 5 (ABHD5/CGI-58) and ATGL to mediate LD lipolysis and lipoprotein morphogenesis.[157] In summary, HCV utilizes the host cell's capacity for lipid metabolism and induces steatosis to complete its life cycle. Hence, in addition to the direct cytopathic effects that result from the virus, the virus leaves in its wake a steatotic program that makes cells susceptible to the aforementioned ER stress.
#
LD Proteins and Genetics of the LD Proteome in HCV
Several perilipin proteins are expressed in the livers of patients with HCV.[39] [158] Among the perilipins, PLIN3 and PLIN5 are upregulated and have divergent roles regarding the protection of LDs during HCV infection. In mice with liver-specific NS5A expression, PLIN5 was found to co-localize with NS5A and protect against NS5A-induced lipolysis and lipotoxic injury.[159] Conversely, in a PLIN3 knockdown mouse study, the core required the presence of PLIN3 to interact with LDs and induce steatosis.[133] In human genetic studies, both PNPLA3 and HSD17B have associations with HCV. In a recent meta-analysis, the PNPLA3 I148M polymorphism increased the risk of fibrosis and cirrhosis by approximately 60% in HCV patients.[160] Finally, in a small case–control study, the HSD17B13 splice variant protects against severe fibrosis in patients infected with HCV[161] and can be used to predict risk of HCC in HCV patients previously treated with direct-acting antivirals.[162]
#
#
Survey of Techniques to Study LDs in Steatosis
Many standard research techniques have been adapted to characterize LDs. Among the major methods, most frequently used by LD researchers are (1) histology and imaging and (2) LD isolation followed by characterization of LD structure and LD composition.
Histology, Imaging, and Quantifying LDs
A primary method to evaluate LDs in hepatocytes and other cells is to fix and stain samples. H and E staining provides a general pathology of LDs in cells such as differences between microsteatosis and macrosteatosis. More specific stains for LDs include BODIPY or LipidSpot610 by fluorescence microscopy[163] [164] [165] [166] [167] and Oil Red O for bright field and fluorescence microscopy.[168] [169] Immunofluorescence assays have been multiplexed to quantify LDs and LD proteins.[163] [164] [168] [170] Co-localization and immunostaining studies were used to initially determine the association and functions of HCV protein's core and NS5A with LDs.[171] To quantify LDs, stained LDs can be counted manually using tools such as Photoshop but this method is quite time-intensive. Several groups have developed more automated software to help quantify light and fluorescent quantification including CellProfiler,[172] Octave,[168] and ImageJ.[169] For example, CellProfiler uses the pixels form a scanned sample to quantify several parameters such as LD number, area, perimeter, and compactness; for a step-by-step guide to analyze and quantify LDs using CellProfiler, we recommend referring to Adomshick et al.[173] These techniques have been applied to LDs in a variety of organisms, cell types, and tissues including liver tissue, hepatocytes, muscle cells, bird oocytes, and yeast. Other visualization techniques used to evaluate LDs include Raman spectroscopy[174] and electron microscopy.[175]
#
LD Isolation and Characterization
The consensus that LDs are metabolically active organelles has led to a surge in LD-specific methods for isolation and characterization. LDs have a low density due to their neutral lipid core allowing researchers to initially separate cell debris and nuclei from less dense cellular components using high-speed centrifugation followed by ultracentrifugation to isolate the floating, white lipid layer on top of the solution. The first method developed was to use density gradient centrifugation with sucrose[176] [177] [178] [179] [180] [181] or Ficoll.[178] Recently, we demonstrated that an organelle isolation kit could be used to separate LDs from other organelles, such as the ER and lysosomes in the mouse liver.[181] This allows direct analysis of LD-associated cellular components within the same samples. After isolation, LDs are washed and fraction purity is checked by western blotting using antibodies that are specific to different subcellular compartments,[182] such as PLIN2 for LDs.
Following isolation and purification of LDs, several methods have been employed to characterize LDs. First, -omics methods have been used to characterize LDs, primarily focused on the LD proteome and lipidome. This includes quantitative mass spectrometry[180] [183] and H[1] NMR[184] [185] [186] for targeted and untargeted lipidomics. Mass spectrometry is also used for quantifying the LD proteome[187] [188]; proximity labeling proteomics has also been used to biotinylate LD proteins for purification followed by mass spectrometry.[189] Additionally, fluorescence assays have been used to assess LD dynamic activities in live cells for interactions with the ER and other organelles[190] and lipolysis.[191] [192] Binding affinities and inter-organelle interactions have been studied in isolated LDs and ERs,[182] and in artificial LDs.[193] [194] Overall, visualization of LDs and characterization of their composition and function have relied on modifications to existing techniques, such as using LD- or LD-protein-specific fluorescent probes and -omics methods.
#
#
Leveraging LD Biology for Therapeutics
Now that more is understood about the role of LDs in the pathogenesis of hepatic steatotic diseases, investigators have leveraged this new knowledge to design therapeutic strategies. The majority of these attempts have been in NASH trials. To date, there have been over 800 clinical trials for NASH and the anticipated market for NASH therapeutics is estimated to be $35 billion by 2025.[195] The emerging role of LD proteins in MASLD pathogenesis provides another opportunity for novel, targeted strategies. For example, for patients who are homozygous for the PNPLA3 risk allele, the drug AZD2693 was developed to specifically lower mRNA expression of PNPLA3 by antisense oligonucleotide technology. A phase I, double-blind, randomized, placebo-controlled, multicenter study was launched in 2020 to administer subcutaneous injections of AZD2693 to NASH patients with F0–F3 fibrosis who are carriers of the PNPLA3 148M risk alleles.[196] While this strategy may be beneficial for steatosis reduction as seen in the antecedent preclinical study,[197] the impact of increased PUFA in the LD fraction that results from ASO administration will need to be better understood given the importance of these lipids on membrane fluidity. There may also be unintended effects as the PNPLA3 polymorphism is expressed in multiple tissues and this strategy would not only target the polymorphism but also wild-type PNPLA3, a protein that is critical for LD homeostasis. Another target launched by the same company, AZD7503, aims to phenocopy the protective effects of the HSD17B splice variant. A single-center, phase I study of this compound in MASLD patients was opened in 2022.[198] Regardless of the eventual results of these particular studies, the integration of genetic diagnostic and therapeutic strategies is likely going to be an integral part of liver disease management for steatotic diseases in the future.
#
Conclusion
LDs and LD proteins are key regulators of cellular lipid homeostasis, and their roles are being elucidated in the pathogenesis of steatotic chronic liver diseases. LDs and their associated proteins limit cellular toxicity associated with bioactive lipids and participate in the regulation of cellular energy. An imbalance in the formation and catabolism of LDs can result from overnutrition and alcohol and can also result from the attempt of viruses to support viral replication. Understanding both the individual functions of LDs and their proteins and the intersection of these proteins with the cellular environment will enable us to fine-tune LD biology in a way that provides better diagnostic and therapeutic solutions for patients at risk for developing or progressing from steatotic liver diseases.
#
#
Conflicts of Interest
R.M.C. receives research support from Merck, Inc, and has consulted for Intercept Pharmaceuticals and Astrazeneca.
-
References
- 1 Chi X, Ogunsade OO, Zhou Z. et al. Lipid droplet is an ancient and inheritable organelle in bacteria. bioRxiv 2020; DOI: 10.1101/2020.05.18.103093.
- 2 Martin S, Parton RG. Lipid droplets: a unified view of a dynamic organelle. Nat Rev Mol Cell Biol 2006; 7 (05) 373-378
- 3 Baselli G, Valenti L. Beyond fat accumulation, NAFLD genetics converges on lipid droplet biology. J Lipid Res 2019; 60 (01) 7-8
- 4 Wilfling F, Wang H, Haas JT. et al. Triacylglycerol synthesis enzymes mediate lipid droplet growth by relocalizing from the ER to lipid droplets. Dev Cell 2013; 24 (04) 384-399
- 5 Stone SJ, Levin MC, Zhou P, Han J, Walther TC, Farese Jr RV. The endoplasmic reticulum enzyme DGAT2 is found in mitochondria-associated membranes and has a mitochondrial targeting signal that promotes its association with mitochondria. J Biol Chem 2009; 284 (08) 5352-5361
- 6 Xu Y, Du X, Turner N, Brown AJ, Yang H. Enhanced acyl-CoA:cholesterol acyltransferase activity increases cholesterol levels on the lipid droplet surface and impairs adipocyte function. J Biol Chem 2019; 294 (50) 19306-19321
- 7 Long T, Sun Y, Hassan A, Qi X, Li X. Structure of nevanimibe-bound tetrameric human ACAT1. Nature 2020; 581 (7808) 339-343
- 8 Santinho A, Salo VT, Chorlay A. et al. Membrane curvature catalyzes lipid droplet assembly. Curr Biol 2020; 30 (13) 2481.e6-2494.e6
- 9 Zoni V, Khaddaj R, Campomanes P, Thiam AR, Schneiter R, Vanni S. Pre-existing bilayer stresses modulate triglyceride accumulation in the ER versus lipid droplets. eLife 2021; 10: 10
- 10 Kim S, Li C, Farese Jr RV, Walther TC, Voth GA. Key factors governing initial stages of lipid droplet formation. J Phys Chem B 2022; 126 (02) 453-462
- 11 Choudhary V, Ojha N, Golden A, Prinz WA. A conserved family of proteins facilitates nascent lipid droplet budding from the ER. J Cell Biol 2015; 211 (02) 261-271
- 12 Arlt H, Sui X, Folger B. et al. Seipin forms a flexible cage at lipid droplet formation sites. Nat Struct Mol Biol 2022; 29 (03) 194-202
- 13 Fei W, Shui G, Gaeta B. et al. Fld1p, a functional homologue of human seipin, regulates the size of lipid droplets in yeast. J Cell Biol 2008; 180 (03) 473-482
- 14 Wang CW, Miao YH, Chang YS. Control of lipid droplet size in budding yeast requires the collaboration between Fld1 and Ldb16. J Cell Sci 2014; 127 (Pt 6): 1214-1228
- 15 Lukmantara I, Chen F, Mak HY. et al. PI(3)P and DFCP1 regulate the biogenesis of lipid droplets. Mol Biol Cell 2022; 33 (14) ar131
- 16 Szymanski KM, Binns D, Bartz R. et al. The lipodystrophy protein seipin is found at endoplasmic reticulum lipid droplet junctions and is important for droplet morphology. Proc Natl Acad Sci U S A 2007; 104 (52) 20890-20895
- 17 Cartwright BR, Binns DD, Hilton CL, Han S, Gao Q, Goodman JM. Seipin performs dissectible functions in promoting lipid droplet biogenesis and regulating droplet morphology. Mol Biol Cell 2015; 26 (04) 726-739
- 18 Correnti J, Lin C, Brettschneider J. et al. Liver-specific ceramide reduction alleviates steatosis and insulin resistance in alcohol-fed mice. J Lipid Res 2020; 61 (07) 983-994
- 19 Williams B, Correnti J, Oranu A. et al. A novel role for ceramide synthase 6 in mouse and human alcoholic steatosis. FASEB J 2018; 32 (01) 130-142
- 20 BasuRay S, Wang Y, Smagris E, Cohen JC, Hobbs HH. Accumulation of PNPLA3 on lipid droplets is the basis of associated hepatic steatosis. Proc Natl Acad Sci U S A 2019; 116 (19) 9521-9526
- 21 Trépo E, Gustot T, Degré D. et al. Common polymorphism in the PNPLA3/adiponutrin gene confers higher risk of cirrhosis and liver damage in alcoholic liver disease. J Hepatol 2011; 55 (04) 906-912
- 22 Stickel F, Buch S, Lau K. et al. Genetic variation in the PNPLA3 gene is associated with alcoholic liver injury in caucasians. Hepatology 2011; 53 (01) 86-95
- 23 Tian C, Stokowski RP, Kershenobich D, Ballinger DG, Hinds DA. Variant in PNPLA3 is associated with alcoholic liver disease. Nat Genet 2010; 42 (01) 21-23
- 24 Dongiovanni P, Donati B, Fares R. et al. PNPLA3 I148M polymorphism and progressive liver disease. World J Gastroenterol 2013; 19 (41) 6969-6978
- 25 Carr RM, Patel RT, Rao V. et al. Reduction of TIP47 improves hepatic steatosis and glucose homeostasis in mice. Am J Physiol Regul Integr Comp Physiol 2012; 302 (08) R996-R1003
- 26 Carr RM, Peralta G, Yin X, Ahima RS. Absence of perilipin 2 prevents hepatic steatosis, glucose intolerance and ceramide accumulation in alcohol-fed mice. PLoS One 2014; 9 (05) e97118
- 27 Varela GM, Antwi DA, Dhir R. et al. Inhibition of ADRP prevents diet-induced insulin resistance. Am J Physiol Gastrointest Liver Physiol 2008; 295 (03) G621-G628
- 28 Verweij N, Haas ME, Nielsen JB. et al. Germline Mutations in CIDEB and Protection against Liver Disease. N Engl J Med 2022; 387 (04) 332-344
- 29 Li JZ, Ye J, Xue B. et al. Cideb regulates diet-induced obesity, liver steatosis, and insulin sensitivity by controlling lipogenesis and fatty acid oxidation. Diabetes 2007; 56 (10) 2523-2532
- 30 Mashek DG, Khan SA, Sathyanarayan A, Ploeger JM, Franklin MP. Hepatic lipid droplet biology: getting to the root of fatty liver. Hepatology 2015; 62 (03) 964-967
- 31 Farese Jr RV, Walther TC. Lipid droplets finally get a little R-E-S-P-E-C-T. Cell 2009; 139 (05) 855-860
- 32 Tandra S, Yeh MM, Brunt EM. et al. Presence and significance of microvesicular steatosis in nonalcoholic fatty liver disease. J Hepatol 2011; 55 (03) 654-659
- 33 Goh GB, McCullough AJ. Natural history of nonalcoholic fatty liver disease. Dig Dis Sci 2016; 61 (05) 1226-1233
- 34 Scorletti E, Carr RM. A new perspective on NAFLD: Focusing on lipid droplets. J Hepatol 2022; 76 (04) 934-945
- 35 Zhang XQ, Xu CF, Yu CH, Chen WX, Li YM. Role of endoplasmic reticulum stress in the pathogenesis of nonalcoholic fatty liver disease. World J Gastroenterol 2014; 20 (07) 1768-1776
- 36 Ben M'barek K, Ajjaji D, Chorlay A, Vanni S, Forêt L, Thiam AR. ER Membrane phospholipids and surface tension control cellular lipid droplet formation. Dev Cell 2017; 41 (06) 591.e7-604.e7
- 37 Song MJ, Malhi H. The unfolded protein response and hepatic lipid metabolism in non alcoholic fatty liver disease. Pharmacol Ther 2019; 203: 107401
- 38 Lebeaupin C, Vallée D, Hazari Y, Hetz C, Chevet E, Bailly-Maitre B. Endoplasmic reticulum stress signalling and the pathogenesis of non-alcoholic fatty liver disease. J Hepatol 2018; 69 (04) 927-947
- 39 Straub BK, Stoeffel P, Heid H, Zimbelmann R, Schirmacher P. Differential pattern of lipid droplet-associated proteins and de novo perilipin expression in hepatocyte steatogenesis. Hepatology 2008; 47 (06) 1936-1946
- 40 Ju L, Han J, Zhang X. et al. Obesity-associated inflammation triggers an autophagy-lysosomal response in adipocytes and causes degradation of perilipin 1. Cell Death Dis 2019; 10 (02) 121
- 41 Carr RM, Dhir R, Mahadev K, Comerford M, Chalasani NP, Ahima RS. Perilipin staining distinguishes between steatosis and nonalcoholic steatohepatitis in adults and children. Clin Gastroenterol Hepatol 2017; 15 (01) 145-147
- 42 Carr RM, Ahima RS. Pathophysiology of lipid droplet proteins in liver diseases. Exp Cell Res 2016; 340 (02) 187-192
- 43 Carr RM, Dhir R, Yin X, Agarwal B, Ahima RS. Temporal effects of ethanol consumption on energy homeostasis, hepatic steatosis, and insulin sensitivity in mice. Alcohol Clin Exp Res 2013; 37 (07) 1091-1099
- 44 McManaman JL, Bales ES, Orlicky DJ. et al. Perilipin-2-null mice are protected against diet-induced obesity, adipose inflammation, and fatty liver disease. J Lipid Res 2013; 54 (05) 1346-1359
- 45 Libby AE, Bales E, Orlicky DJ, McManaman JL. Perilipin-2 deletion impairs hepatic lipid accumulation by interfering with sterol regulatory element-binding protein (SREBP) activation and altering the hepatic lipidome. J Biol Chem 2016; 291 (46) 24231-24246
- 46 Faulkner CS, White CM, Shah VH, Jophlin LL. A single nucleotide polymorphism of PLIN2 is associated with nonalcoholic steatohepatitis and causes phenotypic changes in hepatocyte lipid droplets: a pilot study. Biochim Biophys Acta Mol Cell Biol Lipids 2020; 1865 (05) 158637
- 47 Sentinelli F, Capoccia D, Incani M. et al. The perilipin 2 (PLIN2) gene Ser251Pro missense mutation is associated with reduced insulin secretion and increased insulin sensitivity in Italian obese subjects. Diabetes Metab Res Rev 2016; 32 (06) 550-556
- 48 Magné J, Aminoff A, Perman Sundelin J. et al. The minor allele of the missense polymorphism Ser251Pro in perilipin 2 (PLIN2) disrupts an α-helix, affects lipolysis, and is associated with reduced plasma triglyceride concentration in humans. FASEB J 2013; 27 (08) 3090-3099
- 49 Scorletti E, Vujkovic M, Himes B. et al. PLIN2 rs35568725 POLYMORPHISM AND NON-ALCOHOLIC FATTY LIVER DISEASE: A PHEWAS ANALYSIS. Hepatology 2022; 76: S872
- 50 Nimura S, Yamaguchi T, Ueda K. et al. Olanzapine promotes the accumulation of lipid droplets and the expression of multiple perilipins in human adipocytes. Biochem Biophys Res Commun 2015; 467 (04) 906-912
- 51 Sztalryd C, Brasaemle DL. The perilipin family of lipid droplet proteins: gatekeepers of intracellular lipolysis. Biochim Biophys Acta Mol Cell Biol Lipids 2017; 1862 (10, Pt B): 1221-1232
- 52 Wang C, Zhao Y, Gao X. et al. Perilipin 5 improves hepatic lipotoxicity by inhibiting lipolysis. Hepatology 2015; 61 (03) 870-882
- 53 Asimakopoulou A, Engel KM, Gassler N. et al. Deletion of perilipin 5 protects against hepatic injury in nonalcoholic fatty liver disease via missing inflammasome activation. Cells 2020; 9 (06) 9
- 54 Ma SY, Sun KS, Zhang M. et al. Disruption of Plin5 degradation by CMA causes lipid homeostasis imbalance in NAFLD. Liver Int 2020; 40 (10) 2427-2438
- 55 Keenan SN, Meex RC, Lo JCY. et al. Perilipin 5 deletion in hepatocytes remodels lipid metabolism and causes hepatic insulin resistance in mice. Diabetes 2019; 68 (03) 543-555
- 56 Vujkovic M, Ramdas S, Lorenz KM. et al; Regeneron Genetics Center, Geisinger-Regeneron DiscovEHR Collaboration, EPoS Consortium, VA Million Veteran Program. A multiancestry genome-wide association study of unexplained chronic ALT elevation as a proxy for nonalcoholic fatty liver disease with histological and radiological validation. Nat Genet 2022; 54 (06) 761-771
- 57 Bruschi FV, Claudel T, Tardelli M. et al. The PNPLA3 I148M variant modulates the fibrogenic phenotype of human hepatic stellate cells. Hepatology 2017; 65 (06) 1875-1890
- 58 Romeo S, Kozlitina J, Xing C. et al. Genetic variation in PNPLA3 confers susceptibility to nonalcoholic fatty liver disease. Nat Genet 2008; 40 (12) 1461-1465
- 59 Luukkonen PK, Nick A, Hölttä-Vuori M. et al. Human PNPLA3-I148M variant increases hepatic retention of polyunsaturated fatty acids. JCI Insight 2019; 4 (16) 4
- 60 Verkade HJ, Fast DG, Rusiñol AE, Scraba DG, Vance DE. Impaired biosynthesis of phosphatidylcholine causes a decrease in the number of very low density lipoprotein particles in the Golgi but not in the endoplasmic reticulum of rat liver. J Biol Chem 1993; 268 (33) 24990-24996
- 61 Li Z, Agellon LB, Allen TM. et al. The ratio of phosphatidylcholine to phosphatidylethanolamine influences membrane integrity and steatohepatitis. Cell Metab 2006; 3 (05) 321-331
- 62 Abul-Husn NS, Cheng X, Li AH. et al. A protein-truncating HSD17B13 variant and protection from chronic liver disease. N Engl J Med 2018; 378 (12) 1096-1106
- 63 Luukkonen PK, Tukiainen T, Juuti A. et al. Hydroxysteroid 17-β dehydrogenase 13 variant increases phospholipids and protects against fibrosis in nonalcoholic fatty liver disease. JCI Insight 2020; 5 (05) 5
- 64 Li X, Ye J, Zhou L, Gu W, Fisher EA, Li P. Opposing roles of cell death-inducing DFF45-like effector B and perilipin 2 in controlling hepatic VLDL lipidation. J Lipid Res 2012; 53 (09) 1877-1889
- 65 Xu W, Wu L, Yu M. et al. Differential roles of cell death-inducing DNA fragmentation factor-α-like effector (CIDE) proteins in promoting lipid droplet fusion and growth in subpopulations of hepatocytes. J Biol Chem 2016; 291 (09) 4282-4293
- 66 Ye J, Li JZ, Liu Y. et al. Cideb, an ER- and lipid droplet-associated protein, mediates VLDL lipidation and maturation by interacting with apolipoprotein B. Cell Metab 2009; 9 (02) 177-190
- 67 Brunt EM, Kleiner DE, Wilson LA, Belt P, Neuschwander-Tetri BA. NASH Clinical Research Network (CRN). Nonalcoholic fatty liver disease (NAFLD) activity score and the histopathologic diagnosis in NAFLD: distinct clinicopathologic meanings. Hepatology 2011; 53 (03) 810-820
- 68 Argo CK, Ikura Y, Lackner C, Caldwell SH. The fat droplet in hepatocellular ballooning and implications for scoring nonalcoholic steatohepatitis therapeutic response. Hepatology 2016; 63 (03) 1056-1057
- 69 Fujii H, Ikura Y, Arimoto J. et al. Expression of perilipin and adipophilin in nonalcoholic fatty liver disease; relevance to oxidative injury and hepatocyte ballooning. J Atheroscler Thromb 2009; 16 (06) 893-901
- 70 Caldwell S, Ikura Y, Dias D. et al. Hepatocellular ballooning in NASH. J Hepatol 2010; 53 (04) 719-723
- 71 Marra F, Svegliati-Baroni G. Lipotoxicity and the gut-liver axis in NASH pathogenesis. J Hepatol 2018; 68 (02) 280-295
- 72 Malhi H, Bronk SF, Werneburg NW, Gores GJ. Free fatty acids induce JNK-dependent hepatocyte lipoapoptosis. J Biol Chem 2006; 281 (17) 12093-12101
- 73 Gan LT, Van Rooyen DM, Koina ME, McCuskey RS, Teoh NC, Farrell GC. Hepatocyte free cholesterol lipotoxicity results from JNK1-mediated mitochondrial injury and is HMGB1 and TLR4-dependent. J Hepatol 2014; 61 (06) 1376-1384
- 74 Hager L, Li L, Pun H. et al. Lecithin:cholesterol acyltransferase deficiency protects against cholesterol-induced hepatic endoplasmic reticulum stress in mice. J Biol Chem 2012; 287 (24) 20755-20768
- 75 Francque S, Verrijken A, Mertens I. et al. Noncirrhotic human nonalcoholic fatty liver disease induces portal hypertension in relation to the histological degree of steatosis. Eur J Gastroenterol Hepatol 2010; 22 (12) 1449-1457
- 76 Loneker AE, Alisafaei F, Kant A. et al. Lipid droplets are intracellular mechanical stressors that impair hepatocyte function. Proc Natl Acad Sci U S A 2023; 120 (16) e2216811120
- 77 DeLeve LD. Vascular liver disease and the liver sinusoidal endothelial cell. In: DeLeve LD, Garcia-Tsao G. eds. Vascular Liver Disease: Mechanisms and Management. New York, NY: Springer New York; 2011: 25-40
- 78 Corpechot C, Barbu V, Wendum D. et al. Hypoxia-induced VEGF and collagen I expressions are associated with angiogenesis and fibrogenesis in experimental cirrhosis. Hepatology 2002; 35 (05) 1010-1021
- 79 Seifalian AM, Piasecki C, Agarwal A, Davidson BR. The effect of graded steatosis on flow in the hepatic parenchymal microcirculation. Transplantation 1999; 68 (06) 780-784
- 80 Francque S, Wamutu S, Chatterjee S. et al. Non-alcoholic steatohepatitis induces non-fibrosis-related portal hypertension associated with splanchnic vasodilation and signs of a hyperdynamic circulation in vitro and in vivo in a rat model. Liver Int 2010; 30 (03) 365-375
- 81 Farrell GC, Teoh NC, McCuskey RS. Hepatic microcirculation in fatty liver disease. Anat Rec (Hoboken) 2008; 291 (06) 684-692
- 82 Rockey DC, Bell PD, Hill JA. Fibrosis–a common pathway to organ injury and failure. N Engl J Med 2015; 373 (01) 96
- 83 Sung KC, Wild SH, Byrne CD. Resolution of fatty liver and risk of incident diabetes. J Clin Endocrinol Metab 2013; 98 (09) 3637-3643
- 84 Meyersohn NM, Mayrhofer T, Corey KE. et al. Association of hepatic steatosis with major adverse cardiovascular events, independent of coronary artery disease. Clin Gastroenterol Hepatol 2021; 19 (07) 1480-1488.e14
- 85 Chambergo-Michilot D, Rodrigo-Gallardo PK, Huaman MR, Vasquez-Chavesta AZ, Salinas-Sedo G, Toro-Huamanchumo CJ. Hypertension and histopathology severity of non-alcoholic fatty liver disease among adults with obesity: a cross-sectional study. Clin Exp Gastroenterol 2023; 16: 129-136
- 86 Chin Y, Lim J, Kong G. et al. Hepatic steatosis and advanced hepatic fibrosis are independent predictors of long-term mortality in acute myocardial infarction. Diabetes Obes Metab 2023; 25 (04) 1032-1044
- 87 Spitzer AL, Lao OB, Dick AA. et al. The biopsied donor liver: incorporating macrosteatosis into high-risk donor assessment. Liver Transpl 2010; 16 (07) 874-884
- 88 Asrani SK, Devarbhavi H, Eaton J, Kamath PS. Burden of liver diseases in the world. J Hepatol 2019; 70 (01) 151-171
- 89 Cheemerla S, Balakrishnan M. Global epidemiology of chronic liver disease. Clin Liver Dis (Hoboken) 2021; 17 (05) 365-370
- 90 Jeon S, Carr R. Alcohol effects on hepatic lipid metabolism. J Lipid Res 2020; 61 (04) 470-479
- 91 Schulze RJ, Ding WX. Lipid droplet dynamics in alcoholic fatty liver disease. Liver Res 2019; 3 (3–4): 185-190
- 92 Jeong WI, Osei-Hyiaman D, Park O. et al. Paracrine activation of hepatic CB1 receptors by stellate cell-derived endocannabinoids mediates alcoholic fatty liver. Cell Metab 2008; 7 (03) 227-235
- 93 Esfandiari F, Medici V, Wong DH. et al. Epigenetic regulation of hepatic endoplasmic reticulum stress pathways in the ethanol-fed cystathionine beta synthase-deficient mouse. Hepatology 2010; 51 (03) 932-941
- 94 Dara L, Ji C, Kaplowitz N. The contribution of endoplasmic reticulum stress to liver diseases. Hepatology 2011; 53 (05) 1752-1763
- 95 Peng Z, Borea PA, Varani K. et al. Adenosine signaling contributes to ethanol-induced fatty liver in mice. J Clin Invest 2009; 119 (03) 582-594
- 96 You M, Matsumoto M, Pacold CM, Cho WK, Crabb DW. The role of AMP-activated protein kinase in the action of ethanol in the liver. Gastroenterology 2004; 127 (06) 1798-1808
- 97 You M, Liang X, Ajmo JM, Ness GC. Involvement of mammalian sirtuin 1 in the action of ethanol in the liver. Am J Physiol Gastrointest Liver Physiol 2008; 294 (04) G892-G898
- 98 Horiguchi N, Wang L, Mukhopadhyay P. et al. Cell type-dependent pro- and anti-inflammatory role of signal transducer and activator of transcription 3 in alcoholic liver injury. Gastroenterology 2008; 134 (04) 1148-1158
- 99 Liangpunsakul S, Ross RA, Crabb DW. Activation of carbohydrate response element-binding protein by ethanol. J Investig Med 2013; 61 (02) 270-277
- 100 Marmier S, Dentin R, Daujat-Chavanieu M. et al. Novel role for carbohydrate responsive element binding protein in the control of ethanol metabolism and susceptibility to binge drinking. Hepatology 2015; 62 (04) 1086-1100
- 101 Zhang W, Sun Q, Zhong W, Sun X, Zhou Z. Hepatic peroxisome proliferator-activated receptor gamma signaling contributes to alcohol-induced hepatic steatosis and inflammation in mice. Alcohol Clin Exp Res 2016; 40 (05) 988-999
- 102 Addolorato G, Capristo E, Greco AV, Stefanini GF, Gasbarrini G. Energy expenditure, substrate oxidation, and body composition in subjects with chronic alcoholism: new findings from metabolic assessment. Alcohol Clin Exp Res 1997; 21 (06) 962-967
- 103 Carr RM, Correnti J. Insulin resistance in clinical and experimental alcoholic liver disease. Ann N Y Acad Sci 2015; 1353 (01) 1-20
- 104 Hussain MM, Maxfield FR, Más-Oliva J. et al. Clearance of chylomicron remnants by the low density lipoprotein receptor-related protein/alpha 2-macroglobulin receptor. J Biol Chem 1991; 266 (21) 13936-13940
- 105 Donnelly KL, Smith CI, Schwarzenberg SJ, Jessurun J, Boldt MD, Parks EJ. Sources of fatty acids stored in liver and secreted via lipoproteins in patients with nonalcoholic fatty liver disease. J Clin Invest 2005; 115 (05) 1343-1351
- 106 Mathur M, Yeh YT, Arya RK. et al. Adipose lipolysis is important for ethanol to induce fatty liver in the National Institute on Alcohol Abuse and Alcoholism murine model of chronic and binge ethanol feeding. Hepatology 2023; 77 (05) 1688-1701
- 107 Stahl A, Gimeno RE, Tartaglia LA, Lodish HF. Fatty acid transport proteins: a current view of a growing family. Trends Endocrinol Metab 2001; 12 (06) 266-273
- 108 Zhou J, Febbraio M, Wada T. et al. Hepatic fatty acid transporter Cd36 is a common target of LXR, PXR, and PPARgamma in promoting steatosis. Gastroenterology 2008; 134 (02) 556-567
- 109 Clugston RD, Yuen JJ, Hu Y. et al. CD36-deficient mice are resistant to alcohol- and high-carbohydrate-induced hepatic steatosis. J Lipid Res 2014; 55 (02) 239-246
- 110 Blomstrand R, Kager L, Lantto O. Studies on the ethanol-induced decrease of fatty acid oxidation in rat and human liver slices. Life Sci 1973; 13 (08) 1131-1141
- 111 Cederbaum AI, Lieber CS, Beattie DS, Rubin E. Effect of chronic ethanol ingestion on fatty acid oxidation by hepatic mitochondria. J Biol Chem 1975; 250 (13) 5122-5129
- 112 Hoek JB, Cahill A, Pastorino JG. Alcohol and mitochondria: a dysfunctional relationship. Gastroenterology 2002; 122 (07) 2049-2063
- 113 Correnti JM, Gottshall L, Lin A. et al. Ethanol and C2 ceramide activate fatty acid oxidation in human hepatoma cells. Sci Rep 2018; 8 (01) 12923
- 114 Suter PM, Schutz Y, Jequier E. The effect of ethanol on fat storage in healthy subjects. N Engl J Med 1992; 326 (15) 983-987
- 115 Sonko BJ, Prentice AM, Murgatroyd PR, Goldberg GR, van de Ven ML, Coward WA. Effect of alcohol on postmeal fat storage. Am J Clin Nutr 1994; 59 (03) 619-625
- 116 Sugimoto T, Yamashita S, Ishigami M. et al. Decreased microsomal triglyceride transfer protein activity contributes to initiation of alcoholic liver steatosis in rats. J Hepatol 2002; 36 (02) 157-162
- 117 Nanji AA, Dannenberg AJ, Jokelainen K, Bass NM. Alcoholic liver injury in the rat is associated with reduced expression of peroxisome proliferator-alpha (PPARalpha)-regulated genes and is ameliorated by PPARalpha activation. J Pharmacol Exp Ther 2004; 310 (01) 417-424
- 118 Kharbanda KK, Mailliard ME, Baldwin CR, Beckenhauer HC, Sorrell MF, Tuma DJ. Betaine attenuates alcoholic steatosis by restoring phosphatidylcholine generation via the phosphatidylethanolamine methyltransferase pathway. J Hepatol 2007; 46 (02) 314-321
- 119 Schulze RJ, Rasineni K, Weller SG. et al. Ethanol exposure inhibits hepatocyte lipophagy by inactivating the small guanosine triphosphatase Rab7. Hepatol Commun 2017; 1 (02) 140-152
- 120 Chao X, Wang S, Zhao K. et al. Impaired TFEB-mediated lysosome biogenesis and autophagy promote chronic ethanol-induced liver injury and steatosis in mice. Gastroenterology 2018; 155 (03) 865.e12-879.e12
- 121 Thomes PG, Trambly CS, Fox HS, Tuma DJ, Donohue Jr TM. Acute and chronic ethanol administration differentially modulate hepatic autophagy and transcription factor EB. Alcohol Clin Exp Res 2015; 39 (12) 2354-2363
- 122 Rasineni K, Donohue Jr TM, Thomes PG. et al. Ethanol-induced steatosis involves impairment of lipophagy, associated with reduced Dynamin2 activity. Hepatol Commun 2017; 1 (06) 501-512
- 123 Jambunathan S, Yin J, Khan W, Tamori Y, Puri V. FSP27 promotes lipid droplet clustering and then fusion to regulate triglyceride accumulation. PLoS One 2011; 6 (12) e28614
- 124 Xu MJ, Cai Y, Wang H. et al. Fat-specific protein 27/CIDEC promotes development of alcoholic steatohepatitis in mice and humans. Gastroenterology 2015; 149 (04) 1030.e6-1041.e6
- 125 Gu Y, Yang Y, Cao X. et al. Plin3 protects against alcoholic liver injury by facilitating lipid export from the endoplasmic reticulum. J Cell Biochem 2019; 120 (09) 16075-16087
- 126 Groebner JL, Girón-Bravo MT, Rothberg ML, Adhikari R, Tuma DJ, Tuma PL. Alcohol-induced microtubule acetylation leads to the accumulation of large, immobile lipid droplets. Am J Physiol Gastrointest Liver Physiol 2019; 317 (04) G373-G386
- 127 Buch S, Stickel F, Trépo E. et al. A genome-wide association study confirms PNPLA3 and identifies TM6SF2 and MBOAT7 as risk loci for alcohol-related cirrhosis. Nat Genet 2015; 47 (12) 1443-1448
- 128 Bianco C, Casirati E, Malvestiti F, Valenti L. Genetic predisposition similarities between NASH and ASH: identification of new therapeutic targets. JHEP Rep Innov Hepatol 2021; 3 (03) 100284
- 129 Li JZ, Huang Y, Karaman R. et al. Chronic overexpression of PNPLA3I148M in mouse liver causes hepatic steatosis. J Clin Invest 2012; 122 (11) 4130-4144
- 130 Teo K, Abeysekera KWM, Adams L. et al; EU-PNAFLD Investigators, GOLD Consortium. rs641738C>T near MBOAT7 is associated with liver fat, ALT and fibrosis in NAFLD: a meta-analysis. J Hepatol 2021; 74 (01) 20-30
- 131 Stickel F, Lutz P, Buch S. et al. Genetic variation in HSD17B13 reduces the risk of developing cirrhosis and hepatocellular carcinoma in alcohol misusers. Hepatology 2020; 72 (01) 88-102
- 132 Mohd Hanafiah K, Groeger J, Flaxman AD, Wiersma ST. Global epidemiology of hepatitis C virus infection: new estimates of age-specific antibody to HCV seroprevalence. Hepatology 2013; 57 (04) 1333-1342
- 133 Ferguson D, Zhang J, Davis MA. et al. The lipid droplet-associated protein perilipin 3 facilitates hepatitis C virus-driven hepatic steatosis. J Lipid Res 2017; 58 (02) 420-432
- 134 [Anonymous]. Global progress report on HIV, viral hepatitis and sexually transmitted infections, 2021. In: Accountability for the global health sector strategies 2016–2021: actions for impact. Geneva: World Health Organization; 2021
- 135 Adinolfi LE, Gambardella M, Andreana A, Tripodi MF, Utili R, Ruggiero G. Steatosis accelerates the progression of liver damage of chronic hepatitis C patients and correlates with specific HCV genotype and visceral obesity. Hepatology 2001; 33 (06) 1358-1364
- 136 André P, Komurian-Pradel F, Deforges S. et al. Characterization of low- and very-low-density hepatitis C virus RNA-containing particles. J Virol 2002; 76 (14) 6919-6928
- 137 Niepmann M. Hepatitis C virus RNA translation. Curr Top Microbiol Immunol 2013; 369: 143-166
- 138 McLauchlan J, Lemberg MK, Hope G, Martoglio B. Intramembrane proteolysis promotes trafficking of hepatitis C virus core protein to lipid droplets. EMBO J 2002; 21 (15) 3980-3988
- 139 Boulant S, Montserret R, Hope RG. et al. Structural determinants that target the hepatitis C virus core protein to lipid droplets. J Biol Chem 2006; 281 (31) 22236-22247
- 140 Pène V, Hernandez C, Vauloup-Fellous C, Garaud-Aunis J, Rosenberg AR. Sequential processing of hepatitis C virus core protein by host cell signal peptidase and signal peptide peptidase: a reassessment. J Viral Hepat 2009; 16 (10) 705-715
- 141 Boulant S, Targett-Adams P, McLauchlan J. Disrupting the association of hepatitis C virus core protein with lipid droplets correlates with a loss in production of infectious virus. J Gen Virol 2007; 88 (Pt 8): 2204-2213
- 142 Miyanari Y, Atsuzawa K, Usuda N. et al. The lipid droplet is an important organelle for hepatitis C virus production. Nat Cell Biol 2007; 9 (09) 1089-1097
- 143 Bartenschlager R, Penin F, Lohmann V, André P. Assembly of infectious hepatitis C virus particles. Trends Microbiol 2011; 19 (02) 95-103
- 144 Sidorkiewicz M. Hepatitis C virus uses host lipids to its own advantage. Metabolites 2021; 11 (05) 11
- 145 Gastaminza P, Cheng G, Wieland S, Zhong J, Liao W, Chisari FV. Cellular determinants of hepatitis C virus assembly, maturation, degradation, and secretion. J Virol 2008; 82 (05) 2120-2129
- 146 Hueging K, Doepke M, Vieyres G. et al. Apolipoprotein E codetermines tissue tropism of hepatitis C virus and is crucial for viral cell-to-cell transmission by contributing to a postenvelopment step of assembly. J Virol 2014; 88 (03) 1433-1446
- 147 Lonardo A, Adinolfi LE, Restivo L. et al. Pathogenesis and significance of hepatitis C virus steatosis: an update on survival strategy of a successful pathogen. World J Gastroenterol 2014; 20 (23) 7089-7103
- 148 Hope RG, McLauchlan J. Sequence motifs required for lipid droplet association and protein stability are unique to the hepatitis C virus core protein. J Gen Virol 2000; 81 (Pt 8): 1913-1925
- 149 Loizides-Mangold U, Clément S, Alfonso-Garcia A. et al. HCV 3a core protein increases lipid droplet cholesteryl ester content via a mechanism dependent on sphingolipid biosynthesis. PLoS One 2014; 9 (12) e115309
- 150 Herker E, Harris C, Hernandez C. et al. Efficient hepatitis C virus particle formation requires diacylglycerol acyltransferase-1. Nat Med 2010; 16 (11) 1295-1298
- 151 Camus G, Herker E, Modi AA. et al. Diacylglycerol acyltransferase-1 localizes hepatitis C virus NS5A protein to lipid droplets and enhances NS5A interaction with the viral capsid core. J Biol Chem 2013; 288 (14) 9915-9923
- 152 Xu N, Zhang SO, Cole RA. et al. The FATP1-DGAT2 complex facilitates lipid droplet expansion at the ER-lipid droplet interface. J Cell Biol 2012; 198 (05) 895-911
- 153 Vieyres G, Pietschmann T. HCV pit stop at the lipid droplet: refuel lipids and put on a lipoprotein coat before exit. Cells 2019; 8 (03) 8
- 154 Lerat H, Kammoun HL, Hainault I. et al. Hepatitis C virus proteins induce lipogenesis and defective triglyceride secretion in transgenic mice. J Biol Chem 2009; 284 (48) 33466-33474
- 155 Lowey B, Hertz L, Chiu S. et al. Hepatitis C virus infection induces hepatic expression of NF-κB-inducing kinase and lipogenesis by downregulating miR-122. mBio 2019; 10 (04) e01617-e01619
- 156 Khatun M, Sur S, Steele R, Ray R, Ray RB. Inhibition of long noncoding RNA Linc-Pint by hepatitis C virus in infected hepatocytes enhances lipogenesis. Hepatology 2021; 74 (01) 41-54
- 157 Vieyres G, Reichert I, Carpentier A, Vondran FWR, Pietschmann T. The ATGL lipase cooperates with ABHD5 to mobilize lipids for hepatitis C virus assembly. PLoS Pathog 2020; 16 (06) e1008554
- 158 Carr RM, Dhir R, Mahadev K. et al. Perilipin staining distinguishes between steatosis and non-alcoholic steatohepatitis in adults and children. Clin Gastroenterol Hepatol 2017; 15 (01) 145-147
- 159 Zhang J, Gao X, Yuan Y. et al. Perilipin 5 alleviates HCV NS5A-induced lipotoxic injuries in liver. Lipids Health Dis 2019; 18 (01) 87
- 160 Salameh H, Masadeh M, Al Hanayneh M. et al. PNPLA3 polymorphism increases risk for and severity of chronic hepatitis C liver disease. World J Hepatol 2016; 8 (35) 1584-1592
- 161 About F, Abel L, Cobat A. HCV-associated liver fibrosis and HSD17B13. N Engl J Med 2018; 379 (19) 1875-1876
- 162 Burlone ME, Bellan M, Barbaglia MN. et al. HSD17B13 and other liver fat-modulating genes predict development of hepatocellular carcinoma among HCV-positive cirrhotics with and without viral clearance after DAA treatment. Clin J Gastroenterol 2022; 15 (02) 301-309
- 163 Listenberger LL, Brown DA. Fluorescent detection of lipid droplets and associated proteins. Curr Protoc Cell Biol 2007 ;Chapter 24:Unit 24.22
- 164 Listenberger LL, Studer AM, Brown DA, Wolins NE. Fluorescent detection of lipid droplets and associated proteins. Curr Protoc Cell Biol 2016; 71: 31.1 , 14
- 165 Qiu B, Simon MC. BODIPY 493/503 staining of neutral lipid droplets for microscopy and quantification by flow cytometry. Bio Protoc 2016; 6 (17) 6
- 166 Chen J, Yue F, Kuang S. Labeling and analyzing lipid droplets in mouse muscle stem cells. STAR Protoc 2022; 3 (04) 101849
- 167 Garcia K, Alves A, Ribeiro-Rodrigues TM, Reis F, Viana S. Analysis of fluorescent-stained lipid droplets with 3d reconstruction for hepatic steatosis assessment. J Vis Exp 2023; (196) e65206 DOI: 10.3791/65206.
- 168 Gojanovich AD, Gimenez MC, Masone D. et al. Human adipose-derived mesenchymal stem/stromal cells handling protocols. lipid droplets and proteins double-staining. Front Cell Dev Biol 2018; 6: 33
- 169 Nishad A, Naseem A, Rani S, Malik S. Automated qualitative batch measurement of lipid droplets in the liver of bird using ImageJ. STAR Protoc 2023; 4 (03) 102466
- 170 Liu CY, Zhu J, Xie Z. Visualizing yeast organelles with fluorescent protein markers. J Vis Exp 2022; (182) e63846 DOI: 10.3791/63846.
- 171 Galli A, Scheel TKH, Prentoe JC, Mikkelsen LS, Gottwein JM, Bukh J. Analysis of hepatitis C virus core/NS5A protein co-localization using novel cell culture systems expressing core-NS2 and NS5A of genotypes 1-7. J Gen Virol 2013; 94 (Pt 10): 2221-2235
- 172 Schüssele DS, Haller PK, Haas ML, Hunter C, Sporbeck K, Proikas-Cezanne T. Autophagy profiling in single cells with open source CellProfiler-based image analysis. Autophagy 2023; 19 (01) 338-351
- 173 Adomshick V, Pu Y, Veiga-Lopez A. Automated lipid droplet quantification system for phenotypic analysis of adipocytes using CellProfiler. Toxicol Mech Methods 2020; 30 (05) 378-387
- 174 Stiebing C, Schmölz L, Wallert M, Matthäus C, Lorkowski S, Popp J. Raman imaging of macrophages incubated with triglyceride-enriched oxLDL visualizes translocation of lipids between endocytic vesicles and lipid droplets. J Lipid Res 2017; 58 (05) 876-883
- 175 Fujimoto T, Ohsaki Y, Suzuki M, Cheng J. Imaging lipid droplets by electron microscopy. Methods Cell Biol 2013; 116: 227-251
- 176 Brasaemle DL, Wolins NE. Isolation of lipid droplets from cells by density gradient centrifugation. Curr Protoc Cell Biol 2016; 72: 3.15.1-3.15.13
- 177 Ding Y, Zhang S, Yang L. et al. Isolating lipid droplets from multiple species. Nat Protoc 2013; 8 (01) 43-51
- 178 Mannik J, Meyers A, Dalhaimer P. Isolation of cellular lipid droplets: two purification techniques starting from yeast cells and human placentas. J Vis Exp 2014; (86) 50981 DOI: 10.3791/50981.
- 179 Brasaemle DL, Wolins NE. Isolation of lipid droplets from cells by density gradient centrifugation. Curr Protoc Cell Biol 2016; 72: 15.1 , 13
- 180 Rösch K, Kwiatkowski M, Schlüter H, Herker E. Lipid droplet isolation for quantitative mass spectrometry analysis. J Vis Exp 2017; (122) 55585
- 181 Brettschneider J, Correnti JM, Lin C, Williams B, Oranu A, Kuriakose A, McIver-Jenkins D, Haba A, Kaneza I, Jeon S, Scorletti E, Carr RM. Rapid lipid droplet isolation protocol using a well-established organelle isolation kit. J Vis Exp 2019; (146) e59290 DOI: 10.3791/59290.
- 182 Kamerkar S, Singh J, Tripathy S, Bhonsle H, Kumar M, Mallik R. Metabolic and immune-sensitive contacts between lipid droplets and endoplasmic reticulum reconstituted in vitro. Proc Natl Acad Sci U S A 2022; 119 (24) e2200513119
- 183 Preuss C, Jelenik T, Bódis K. et al. A new targeted lipidomics approach reveals lipid droplets in liver, muscle and heart as a repository for diacylglycerol and ceramide species in non-alcoholic fatty liver. Cells 2019; 8 (03) 8
- 184 Rémy C, Fouilhé N, Barba I. et al. Evidence that mobile lipids detected in rat brain glioma by 1H nuclear magnetic resonance correspond to lipid droplets. Cancer Res 1997; 57 (03) 407-414
- 185 Bartz R, Li WH, Venables B. et al. Lipidomics reveals that adiposomes store ether lipids and mediate phospholipid traffic. J Lipid Res 2007; 48 (04) 837-847
- 186 Nigam S, Ranjan R, Sinha N, Ateeq B. Nuclear magnetic resonance spectroscopy reveals dysregulation of monounsaturated fatty acid metabolism upon SPINK1 attenuation in colorectal cancer. NMR Biomed 2022; 35 (07) e4705
- 187 Schmidt C, Ploier B, Koch B, Daum G. Analysis of yeast lipid droplet proteome and lipidome. Methods Cell Biol 2013; 116: 15-37
- 188 Lagrutta LC, Layerenza JP, Bronsoms S, Trejo SA, Ves-Losada A. Nuclear-lipid-droplet proteome: carboxylesterase as a nuclear lipase involved in lipid-droplet homeostasis. Heliyon 2021; 7 (03) e06539
- 189 Peterson CWH, Deol KK, To M, Olzmann JA. Optimized protocol for the identification of lipid droplet proteomes using proximity labeling proteomics in cultured human cells. STAR Protoc 2021; 2 (02) 100579
- 190 Tatenaka Y, Kato H, Ishiyama M. et al. Monitoring lipid droplet dynamics in living cells by using fluorescent probes. Biochemistry 2019; 58 (06) 499-503
- 191 Ma X, Zhi Z, Zhang S, Liu P. Measurement of ATGL activity using adiposomes. Biophys Rep 2023; 9 (01) 3-14
- 192 Bridge-Comer PE, Reilly SM. Measuring the rate of lipolysis in ex vivo murine adipose tissue and primary preadipocytes differentiated in vitro. J Vis Exp 2023; (193) e65106 DOI: 10.3791/65106.
- 193 Chorlay A, Santinho A, Thiam AR. Making droplet-embedded vesicles to model cellular lipid droplets. STAR Protoc 2020; 1 (03) 100116
- 194 Zhi Z, Ma X, Zhou C, Mechler A, Zhang S, Liu P. Protocol for using artificial lipid droplets to study the binding affinity of lipid droplet-associated proteins. STAR Protoc 2022; 3 (01) 101214
- 195 Drew L. Drug development: sprint finish. Nature 2017; 551 (7681) 551
- 196 A study to assess safety, tolerability, PK and PD of AZD2693 in non-alcoholic steatohepatitis patients. Accessed September 25, 2023, at: https://clinicaltrials.gov/ct2/show/NCT04483947
- 197 Lindén D, Ahnmark A, Pingitore P. et al. Pnpla3 silencing with antisense oligonucleotides ameliorates nonalcoholic steatohepatitis and fibrosis in Pnpla3 I148M knock-in mice. Mol Metab 2019; 22: 49-61
- 198 Knockdown of HSD17B13 mRNA, pharmacokinetics, safety, and tolerability, of AZD7503 in non-alcoholic fatty liver disease. Accessed September 25, 2023, at: https://clinicaltrials.gov/ct2/show/NCT05560607
Address for correspondence
Publication History
Accepted Manuscript online:
05 October 2023
Article published online:
06 November 2023
© 2023. Thieme. All rights reserved.
Thieme Medical Publishers, Inc.
333 Seventh Avenue, 18th Floor, New York, NY 10001, USA
-
References
- 1 Chi X, Ogunsade OO, Zhou Z. et al. Lipid droplet is an ancient and inheritable organelle in bacteria. bioRxiv 2020; DOI: 10.1101/2020.05.18.103093.
- 2 Martin S, Parton RG. Lipid droplets: a unified view of a dynamic organelle. Nat Rev Mol Cell Biol 2006; 7 (05) 373-378
- 3 Baselli G, Valenti L. Beyond fat accumulation, NAFLD genetics converges on lipid droplet biology. J Lipid Res 2019; 60 (01) 7-8
- 4 Wilfling F, Wang H, Haas JT. et al. Triacylglycerol synthesis enzymes mediate lipid droplet growth by relocalizing from the ER to lipid droplets. Dev Cell 2013; 24 (04) 384-399
- 5 Stone SJ, Levin MC, Zhou P, Han J, Walther TC, Farese Jr RV. The endoplasmic reticulum enzyme DGAT2 is found in mitochondria-associated membranes and has a mitochondrial targeting signal that promotes its association with mitochondria. J Biol Chem 2009; 284 (08) 5352-5361
- 6 Xu Y, Du X, Turner N, Brown AJ, Yang H. Enhanced acyl-CoA:cholesterol acyltransferase activity increases cholesterol levels on the lipid droplet surface and impairs adipocyte function. J Biol Chem 2019; 294 (50) 19306-19321
- 7 Long T, Sun Y, Hassan A, Qi X, Li X. Structure of nevanimibe-bound tetrameric human ACAT1. Nature 2020; 581 (7808) 339-343
- 8 Santinho A, Salo VT, Chorlay A. et al. Membrane curvature catalyzes lipid droplet assembly. Curr Biol 2020; 30 (13) 2481.e6-2494.e6
- 9 Zoni V, Khaddaj R, Campomanes P, Thiam AR, Schneiter R, Vanni S. Pre-existing bilayer stresses modulate triglyceride accumulation in the ER versus lipid droplets. eLife 2021; 10: 10
- 10 Kim S, Li C, Farese Jr RV, Walther TC, Voth GA. Key factors governing initial stages of lipid droplet formation. J Phys Chem B 2022; 126 (02) 453-462
- 11 Choudhary V, Ojha N, Golden A, Prinz WA. A conserved family of proteins facilitates nascent lipid droplet budding from the ER. J Cell Biol 2015; 211 (02) 261-271
- 12 Arlt H, Sui X, Folger B. et al. Seipin forms a flexible cage at lipid droplet formation sites. Nat Struct Mol Biol 2022; 29 (03) 194-202
- 13 Fei W, Shui G, Gaeta B. et al. Fld1p, a functional homologue of human seipin, regulates the size of lipid droplets in yeast. J Cell Biol 2008; 180 (03) 473-482
- 14 Wang CW, Miao YH, Chang YS. Control of lipid droplet size in budding yeast requires the collaboration between Fld1 and Ldb16. J Cell Sci 2014; 127 (Pt 6): 1214-1228
- 15 Lukmantara I, Chen F, Mak HY. et al. PI(3)P and DFCP1 regulate the biogenesis of lipid droplets. Mol Biol Cell 2022; 33 (14) ar131
- 16 Szymanski KM, Binns D, Bartz R. et al. The lipodystrophy protein seipin is found at endoplasmic reticulum lipid droplet junctions and is important for droplet morphology. Proc Natl Acad Sci U S A 2007; 104 (52) 20890-20895
- 17 Cartwright BR, Binns DD, Hilton CL, Han S, Gao Q, Goodman JM. Seipin performs dissectible functions in promoting lipid droplet biogenesis and regulating droplet morphology. Mol Biol Cell 2015; 26 (04) 726-739
- 18 Correnti J, Lin C, Brettschneider J. et al. Liver-specific ceramide reduction alleviates steatosis and insulin resistance in alcohol-fed mice. J Lipid Res 2020; 61 (07) 983-994
- 19 Williams B, Correnti J, Oranu A. et al. A novel role for ceramide synthase 6 in mouse and human alcoholic steatosis. FASEB J 2018; 32 (01) 130-142
- 20 BasuRay S, Wang Y, Smagris E, Cohen JC, Hobbs HH. Accumulation of PNPLA3 on lipid droplets is the basis of associated hepatic steatosis. Proc Natl Acad Sci U S A 2019; 116 (19) 9521-9526
- 21 Trépo E, Gustot T, Degré D. et al. Common polymorphism in the PNPLA3/adiponutrin gene confers higher risk of cirrhosis and liver damage in alcoholic liver disease. J Hepatol 2011; 55 (04) 906-912
- 22 Stickel F, Buch S, Lau K. et al. Genetic variation in the PNPLA3 gene is associated with alcoholic liver injury in caucasians. Hepatology 2011; 53 (01) 86-95
- 23 Tian C, Stokowski RP, Kershenobich D, Ballinger DG, Hinds DA. Variant in PNPLA3 is associated with alcoholic liver disease. Nat Genet 2010; 42 (01) 21-23
- 24 Dongiovanni P, Donati B, Fares R. et al. PNPLA3 I148M polymorphism and progressive liver disease. World J Gastroenterol 2013; 19 (41) 6969-6978
- 25 Carr RM, Patel RT, Rao V. et al. Reduction of TIP47 improves hepatic steatosis and glucose homeostasis in mice. Am J Physiol Regul Integr Comp Physiol 2012; 302 (08) R996-R1003
- 26 Carr RM, Peralta G, Yin X, Ahima RS. Absence of perilipin 2 prevents hepatic steatosis, glucose intolerance and ceramide accumulation in alcohol-fed mice. PLoS One 2014; 9 (05) e97118
- 27 Varela GM, Antwi DA, Dhir R. et al. Inhibition of ADRP prevents diet-induced insulin resistance. Am J Physiol Gastrointest Liver Physiol 2008; 295 (03) G621-G628
- 28 Verweij N, Haas ME, Nielsen JB. et al. Germline Mutations in CIDEB and Protection against Liver Disease. N Engl J Med 2022; 387 (04) 332-344
- 29 Li JZ, Ye J, Xue B. et al. Cideb regulates diet-induced obesity, liver steatosis, and insulin sensitivity by controlling lipogenesis and fatty acid oxidation. Diabetes 2007; 56 (10) 2523-2532
- 30 Mashek DG, Khan SA, Sathyanarayan A, Ploeger JM, Franklin MP. Hepatic lipid droplet biology: getting to the root of fatty liver. Hepatology 2015; 62 (03) 964-967
- 31 Farese Jr RV, Walther TC. Lipid droplets finally get a little R-E-S-P-E-C-T. Cell 2009; 139 (05) 855-860
- 32 Tandra S, Yeh MM, Brunt EM. et al. Presence and significance of microvesicular steatosis in nonalcoholic fatty liver disease. J Hepatol 2011; 55 (03) 654-659
- 33 Goh GB, McCullough AJ. Natural history of nonalcoholic fatty liver disease. Dig Dis Sci 2016; 61 (05) 1226-1233
- 34 Scorletti E, Carr RM. A new perspective on NAFLD: Focusing on lipid droplets. J Hepatol 2022; 76 (04) 934-945
- 35 Zhang XQ, Xu CF, Yu CH, Chen WX, Li YM. Role of endoplasmic reticulum stress in the pathogenesis of nonalcoholic fatty liver disease. World J Gastroenterol 2014; 20 (07) 1768-1776
- 36 Ben M'barek K, Ajjaji D, Chorlay A, Vanni S, Forêt L, Thiam AR. ER Membrane phospholipids and surface tension control cellular lipid droplet formation. Dev Cell 2017; 41 (06) 591.e7-604.e7
- 37 Song MJ, Malhi H. The unfolded protein response and hepatic lipid metabolism in non alcoholic fatty liver disease. Pharmacol Ther 2019; 203: 107401
- 38 Lebeaupin C, Vallée D, Hazari Y, Hetz C, Chevet E, Bailly-Maitre B. Endoplasmic reticulum stress signalling and the pathogenesis of non-alcoholic fatty liver disease. J Hepatol 2018; 69 (04) 927-947
- 39 Straub BK, Stoeffel P, Heid H, Zimbelmann R, Schirmacher P. Differential pattern of lipid droplet-associated proteins and de novo perilipin expression in hepatocyte steatogenesis. Hepatology 2008; 47 (06) 1936-1946
- 40 Ju L, Han J, Zhang X. et al. Obesity-associated inflammation triggers an autophagy-lysosomal response in adipocytes and causes degradation of perilipin 1. Cell Death Dis 2019; 10 (02) 121
- 41 Carr RM, Dhir R, Mahadev K, Comerford M, Chalasani NP, Ahima RS. Perilipin staining distinguishes between steatosis and nonalcoholic steatohepatitis in adults and children. Clin Gastroenterol Hepatol 2017; 15 (01) 145-147
- 42 Carr RM, Ahima RS. Pathophysiology of lipid droplet proteins in liver diseases. Exp Cell Res 2016; 340 (02) 187-192
- 43 Carr RM, Dhir R, Yin X, Agarwal B, Ahima RS. Temporal effects of ethanol consumption on energy homeostasis, hepatic steatosis, and insulin sensitivity in mice. Alcohol Clin Exp Res 2013; 37 (07) 1091-1099
- 44 McManaman JL, Bales ES, Orlicky DJ. et al. Perilipin-2-null mice are protected against diet-induced obesity, adipose inflammation, and fatty liver disease. J Lipid Res 2013; 54 (05) 1346-1359
- 45 Libby AE, Bales E, Orlicky DJ, McManaman JL. Perilipin-2 deletion impairs hepatic lipid accumulation by interfering with sterol regulatory element-binding protein (SREBP) activation and altering the hepatic lipidome. J Biol Chem 2016; 291 (46) 24231-24246
- 46 Faulkner CS, White CM, Shah VH, Jophlin LL. A single nucleotide polymorphism of PLIN2 is associated with nonalcoholic steatohepatitis and causes phenotypic changes in hepatocyte lipid droplets: a pilot study. Biochim Biophys Acta Mol Cell Biol Lipids 2020; 1865 (05) 158637
- 47 Sentinelli F, Capoccia D, Incani M. et al. The perilipin 2 (PLIN2) gene Ser251Pro missense mutation is associated with reduced insulin secretion and increased insulin sensitivity in Italian obese subjects. Diabetes Metab Res Rev 2016; 32 (06) 550-556
- 48 Magné J, Aminoff A, Perman Sundelin J. et al. The minor allele of the missense polymorphism Ser251Pro in perilipin 2 (PLIN2) disrupts an α-helix, affects lipolysis, and is associated with reduced plasma triglyceride concentration in humans. FASEB J 2013; 27 (08) 3090-3099
- 49 Scorletti E, Vujkovic M, Himes B. et al. PLIN2 rs35568725 POLYMORPHISM AND NON-ALCOHOLIC FATTY LIVER DISEASE: A PHEWAS ANALYSIS. Hepatology 2022; 76: S872
- 50 Nimura S, Yamaguchi T, Ueda K. et al. Olanzapine promotes the accumulation of lipid droplets and the expression of multiple perilipins in human adipocytes. Biochem Biophys Res Commun 2015; 467 (04) 906-912
- 51 Sztalryd C, Brasaemle DL. The perilipin family of lipid droplet proteins: gatekeepers of intracellular lipolysis. Biochim Biophys Acta Mol Cell Biol Lipids 2017; 1862 (10, Pt B): 1221-1232
- 52 Wang C, Zhao Y, Gao X. et al. Perilipin 5 improves hepatic lipotoxicity by inhibiting lipolysis. Hepatology 2015; 61 (03) 870-882
- 53 Asimakopoulou A, Engel KM, Gassler N. et al. Deletion of perilipin 5 protects against hepatic injury in nonalcoholic fatty liver disease via missing inflammasome activation. Cells 2020; 9 (06) 9
- 54 Ma SY, Sun KS, Zhang M. et al. Disruption of Plin5 degradation by CMA causes lipid homeostasis imbalance in NAFLD. Liver Int 2020; 40 (10) 2427-2438
- 55 Keenan SN, Meex RC, Lo JCY. et al. Perilipin 5 deletion in hepatocytes remodels lipid metabolism and causes hepatic insulin resistance in mice. Diabetes 2019; 68 (03) 543-555
- 56 Vujkovic M, Ramdas S, Lorenz KM. et al; Regeneron Genetics Center, Geisinger-Regeneron DiscovEHR Collaboration, EPoS Consortium, VA Million Veteran Program. A multiancestry genome-wide association study of unexplained chronic ALT elevation as a proxy for nonalcoholic fatty liver disease with histological and radiological validation. Nat Genet 2022; 54 (06) 761-771
- 57 Bruschi FV, Claudel T, Tardelli M. et al. The PNPLA3 I148M variant modulates the fibrogenic phenotype of human hepatic stellate cells. Hepatology 2017; 65 (06) 1875-1890
- 58 Romeo S, Kozlitina J, Xing C. et al. Genetic variation in PNPLA3 confers susceptibility to nonalcoholic fatty liver disease. Nat Genet 2008; 40 (12) 1461-1465
- 59 Luukkonen PK, Nick A, Hölttä-Vuori M. et al. Human PNPLA3-I148M variant increases hepatic retention of polyunsaturated fatty acids. JCI Insight 2019; 4 (16) 4
- 60 Verkade HJ, Fast DG, Rusiñol AE, Scraba DG, Vance DE. Impaired biosynthesis of phosphatidylcholine causes a decrease in the number of very low density lipoprotein particles in the Golgi but not in the endoplasmic reticulum of rat liver. J Biol Chem 1993; 268 (33) 24990-24996
- 61 Li Z, Agellon LB, Allen TM. et al. The ratio of phosphatidylcholine to phosphatidylethanolamine influences membrane integrity and steatohepatitis. Cell Metab 2006; 3 (05) 321-331
- 62 Abul-Husn NS, Cheng X, Li AH. et al. A protein-truncating HSD17B13 variant and protection from chronic liver disease. N Engl J Med 2018; 378 (12) 1096-1106
- 63 Luukkonen PK, Tukiainen T, Juuti A. et al. Hydroxysteroid 17-β dehydrogenase 13 variant increases phospholipids and protects against fibrosis in nonalcoholic fatty liver disease. JCI Insight 2020; 5 (05) 5
- 64 Li X, Ye J, Zhou L, Gu W, Fisher EA, Li P. Opposing roles of cell death-inducing DFF45-like effector B and perilipin 2 in controlling hepatic VLDL lipidation. J Lipid Res 2012; 53 (09) 1877-1889
- 65 Xu W, Wu L, Yu M. et al. Differential roles of cell death-inducing DNA fragmentation factor-α-like effector (CIDE) proteins in promoting lipid droplet fusion and growth in subpopulations of hepatocytes. J Biol Chem 2016; 291 (09) 4282-4293
- 66 Ye J, Li JZ, Liu Y. et al. Cideb, an ER- and lipid droplet-associated protein, mediates VLDL lipidation and maturation by interacting with apolipoprotein B. Cell Metab 2009; 9 (02) 177-190
- 67 Brunt EM, Kleiner DE, Wilson LA, Belt P, Neuschwander-Tetri BA. NASH Clinical Research Network (CRN). Nonalcoholic fatty liver disease (NAFLD) activity score and the histopathologic diagnosis in NAFLD: distinct clinicopathologic meanings. Hepatology 2011; 53 (03) 810-820
- 68 Argo CK, Ikura Y, Lackner C, Caldwell SH. The fat droplet in hepatocellular ballooning and implications for scoring nonalcoholic steatohepatitis therapeutic response. Hepatology 2016; 63 (03) 1056-1057
- 69 Fujii H, Ikura Y, Arimoto J. et al. Expression of perilipin and adipophilin in nonalcoholic fatty liver disease; relevance to oxidative injury and hepatocyte ballooning. J Atheroscler Thromb 2009; 16 (06) 893-901
- 70 Caldwell S, Ikura Y, Dias D. et al. Hepatocellular ballooning in NASH. J Hepatol 2010; 53 (04) 719-723
- 71 Marra F, Svegliati-Baroni G. Lipotoxicity and the gut-liver axis in NASH pathogenesis. J Hepatol 2018; 68 (02) 280-295
- 72 Malhi H, Bronk SF, Werneburg NW, Gores GJ. Free fatty acids induce JNK-dependent hepatocyte lipoapoptosis. J Biol Chem 2006; 281 (17) 12093-12101
- 73 Gan LT, Van Rooyen DM, Koina ME, McCuskey RS, Teoh NC, Farrell GC. Hepatocyte free cholesterol lipotoxicity results from JNK1-mediated mitochondrial injury and is HMGB1 and TLR4-dependent. J Hepatol 2014; 61 (06) 1376-1384
- 74 Hager L, Li L, Pun H. et al. Lecithin:cholesterol acyltransferase deficiency protects against cholesterol-induced hepatic endoplasmic reticulum stress in mice. J Biol Chem 2012; 287 (24) 20755-20768
- 75 Francque S, Verrijken A, Mertens I. et al. Noncirrhotic human nonalcoholic fatty liver disease induces portal hypertension in relation to the histological degree of steatosis. Eur J Gastroenterol Hepatol 2010; 22 (12) 1449-1457
- 76 Loneker AE, Alisafaei F, Kant A. et al. Lipid droplets are intracellular mechanical stressors that impair hepatocyte function. Proc Natl Acad Sci U S A 2023; 120 (16) e2216811120
- 77 DeLeve LD. Vascular liver disease and the liver sinusoidal endothelial cell. In: DeLeve LD, Garcia-Tsao G. eds. Vascular Liver Disease: Mechanisms and Management. New York, NY: Springer New York; 2011: 25-40
- 78 Corpechot C, Barbu V, Wendum D. et al. Hypoxia-induced VEGF and collagen I expressions are associated with angiogenesis and fibrogenesis in experimental cirrhosis. Hepatology 2002; 35 (05) 1010-1021
- 79 Seifalian AM, Piasecki C, Agarwal A, Davidson BR. The effect of graded steatosis on flow in the hepatic parenchymal microcirculation. Transplantation 1999; 68 (06) 780-784
- 80 Francque S, Wamutu S, Chatterjee S. et al. Non-alcoholic steatohepatitis induces non-fibrosis-related portal hypertension associated with splanchnic vasodilation and signs of a hyperdynamic circulation in vitro and in vivo in a rat model. Liver Int 2010; 30 (03) 365-375
- 81 Farrell GC, Teoh NC, McCuskey RS. Hepatic microcirculation in fatty liver disease. Anat Rec (Hoboken) 2008; 291 (06) 684-692
- 82 Rockey DC, Bell PD, Hill JA. Fibrosis–a common pathway to organ injury and failure. N Engl J Med 2015; 373 (01) 96
- 83 Sung KC, Wild SH, Byrne CD. Resolution of fatty liver and risk of incident diabetes. J Clin Endocrinol Metab 2013; 98 (09) 3637-3643
- 84 Meyersohn NM, Mayrhofer T, Corey KE. et al. Association of hepatic steatosis with major adverse cardiovascular events, independent of coronary artery disease. Clin Gastroenterol Hepatol 2021; 19 (07) 1480-1488.e14
- 85 Chambergo-Michilot D, Rodrigo-Gallardo PK, Huaman MR, Vasquez-Chavesta AZ, Salinas-Sedo G, Toro-Huamanchumo CJ. Hypertension and histopathology severity of non-alcoholic fatty liver disease among adults with obesity: a cross-sectional study. Clin Exp Gastroenterol 2023; 16: 129-136
- 86 Chin Y, Lim J, Kong G. et al. Hepatic steatosis and advanced hepatic fibrosis are independent predictors of long-term mortality in acute myocardial infarction. Diabetes Obes Metab 2023; 25 (04) 1032-1044
- 87 Spitzer AL, Lao OB, Dick AA. et al. The biopsied donor liver: incorporating macrosteatosis into high-risk donor assessment. Liver Transpl 2010; 16 (07) 874-884
- 88 Asrani SK, Devarbhavi H, Eaton J, Kamath PS. Burden of liver diseases in the world. J Hepatol 2019; 70 (01) 151-171
- 89 Cheemerla S, Balakrishnan M. Global epidemiology of chronic liver disease. Clin Liver Dis (Hoboken) 2021; 17 (05) 365-370
- 90 Jeon S, Carr R. Alcohol effects on hepatic lipid metabolism. J Lipid Res 2020; 61 (04) 470-479
- 91 Schulze RJ, Ding WX. Lipid droplet dynamics in alcoholic fatty liver disease. Liver Res 2019; 3 (3–4): 185-190
- 92 Jeong WI, Osei-Hyiaman D, Park O. et al. Paracrine activation of hepatic CB1 receptors by stellate cell-derived endocannabinoids mediates alcoholic fatty liver. Cell Metab 2008; 7 (03) 227-235
- 93 Esfandiari F, Medici V, Wong DH. et al. Epigenetic regulation of hepatic endoplasmic reticulum stress pathways in the ethanol-fed cystathionine beta synthase-deficient mouse. Hepatology 2010; 51 (03) 932-941
- 94 Dara L, Ji C, Kaplowitz N. The contribution of endoplasmic reticulum stress to liver diseases. Hepatology 2011; 53 (05) 1752-1763
- 95 Peng Z, Borea PA, Varani K. et al. Adenosine signaling contributes to ethanol-induced fatty liver in mice. J Clin Invest 2009; 119 (03) 582-594
- 96 You M, Matsumoto M, Pacold CM, Cho WK, Crabb DW. The role of AMP-activated protein kinase in the action of ethanol in the liver. Gastroenterology 2004; 127 (06) 1798-1808
- 97 You M, Liang X, Ajmo JM, Ness GC. Involvement of mammalian sirtuin 1 in the action of ethanol in the liver. Am J Physiol Gastrointest Liver Physiol 2008; 294 (04) G892-G898
- 98 Horiguchi N, Wang L, Mukhopadhyay P. et al. Cell type-dependent pro- and anti-inflammatory role of signal transducer and activator of transcription 3 in alcoholic liver injury. Gastroenterology 2008; 134 (04) 1148-1158
- 99 Liangpunsakul S, Ross RA, Crabb DW. Activation of carbohydrate response element-binding protein by ethanol. J Investig Med 2013; 61 (02) 270-277
- 100 Marmier S, Dentin R, Daujat-Chavanieu M. et al. Novel role for carbohydrate responsive element binding protein in the control of ethanol metabolism and susceptibility to binge drinking. Hepatology 2015; 62 (04) 1086-1100
- 101 Zhang W, Sun Q, Zhong W, Sun X, Zhou Z. Hepatic peroxisome proliferator-activated receptor gamma signaling contributes to alcohol-induced hepatic steatosis and inflammation in mice. Alcohol Clin Exp Res 2016; 40 (05) 988-999
- 102 Addolorato G, Capristo E, Greco AV, Stefanini GF, Gasbarrini G. Energy expenditure, substrate oxidation, and body composition in subjects with chronic alcoholism: new findings from metabolic assessment. Alcohol Clin Exp Res 1997; 21 (06) 962-967
- 103 Carr RM, Correnti J. Insulin resistance in clinical and experimental alcoholic liver disease. Ann N Y Acad Sci 2015; 1353 (01) 1-20
- 104 Hussain MM, Maxfield FR, Más-Oliva J. et al. Clearance of chylomicron remnants by the low density lipoprotein receptor-related protein/alpha 2-macroglobulin receptor. J Biol Chem 1991; 266 (21) 13936-13940
- 105 Donnelly KL, Smith CI, Schwarzenberg SJ, Jessurun J, Boldt MD, Parks EJ. Sources of fatty acids stored in liver and secreted via lipoproteins in patients with nonalcoholic fatty liver disease. J Clin Invest 2005; 115 (05) 1343-1351
- 106 Mathur M, Yeh YT, Arya RK. et al. Adipose lipolysis is important for ethanol to induce fatty liver in the National Institute on Alcohol Abuse and Alcoholism murine model of chronic and binge ethanol feeding. Hepatology 2023; 77 (05) 1688-1701
- 107 Stahl A, Gimeno RE, Tartaglia LA, Lodish HF. Fatty acid transport proteins: a current view of a growing family. Trends Endocrinol Metab 2001; 12 (06) 266-273
- 108 Zhou J, Febbraio M, Wada T. et al. Hepatic fatty acid transporter Cd36 is a common target of LXR, PXR, and PPARgamma in promoting steatosis. Gastroenterology 2008; 134 (02) 556-567
- 109 Clugston RD, Yuen JJ, Hu Y. et al. CD36-deficient mice are resistant to alcohol- and high-carbohydrate-induced hepatic steatosis. J Lipid Res 2014; 55 (02) 239-246
- 110 Blomstrand R, Kager L, Lantto O. Studies on the ethanol-induced decrease of fatty acid oxidation in rat and human liver slices. Life Sci 1973; 13 (08) 1131-1141
- 111 Cederbaum AI, Lieber CS, Beattie DS, Rubin E. Effect of chronic ethanol ingestion on fatty acid oxidation by hepatic mitochondria. J Biol Chem 1975; 250 (13) 5122-5129
- 112 Hoek JB, Cahill A, Pastorino JG. Alcohol and mitochondria: a dysfunctional relationship. Gastroenterology 2002; 122 (07) 2049-2063
- 113 Correnti JM, Gottshall L, Lin A. et al. Ethanol and C2 ceramide activate fatty acid oxidation in human hepatoma cells. Sci Rep 2018; 8 (01) 12923
- 114 Suter PM, Schutz Y, Jequier E. The effect of ethanol on fat storage in healthy subjects. N Engl J Med 1992; 326 (15) 983-987
- 115 Sonko BJ, Prentice AM, Murgatroyd PR, Goldberg GR, van de Ven ML, Coward WA. Effect of alcohol on postmeal fat storage. Am J Clin Nutr 1994; 59 (03) 619-625
- 116 Sugimoto T, Yamashita S, Ishigami M. et al. Decreased microsomal triglyceride transfer protein activity contributes to initiation of alcoholic liver steatosis in rats. J Hepatol 2002; 36 (02) 157-162
- 117 Nanji AA, Dannenberg AJ, Jokelainen K, Bass NM. Alcoholic liver injury in the rat is associated with reduced expression of peroxisome proliferator-alpha (PPARalpha)-regulated genes and is ameliorated by PPARalpha activation. J Pharmacol Exp Ther 2004; 310 (01) 417-424
- 118 Kharbanda KK, Mailliard ME, Baldwin CR, Beckenhauer HC, Sorrell MF, Tuma DJ. Betaine attenuates alcoholic steatosis by restoring phosphatidylcholine generation via the phosphatidylethanolamine methyltransferase pathway. J Hepatol 2007; 46 (02) 314-321
- 119 Schulze RJ, Rasineni K, Weller SG. et al. Ethanol exposure inhibits hepatocyte lipophagy by inactivating the small guanosine triphosphatase Rab7. Hepatol Commun 2017; 1 (02) 140-152
- 120 Chao X, Wang S, Zhao K. et al. Impaired TFEB-mediated lysosome biogenesis and autophagy promote chronic ethanol-induced liver injury and steatosis in mice. Gastroenterology 2018; 155 (03) 865.e12-879.e12
- 121 Thomes PG, Trambly CS, Fox HS, Tuma DJ, Donohue Jr TM. Acute and chronic ethanol administration differentially modulate hepatic autophagy and transcription factor EB. Alcohol Clin Exp Res 2015; 39 (12) 2354-2363
- 122 Rasineni K, Donohue Jr TM, Thomes PG. et al. Ethanol-induced steatosis involves impairment of lipophagy, associated with reduced Dynamin2 activity. Hepatol Commun 2017; 1 (06) 501-512
- 123 Jambunathan S, Yin J, Khan W, Tamori Y, Puri V. FSP27 promotes lipid droplet clustering and then fusion to regulate triglyceride accumulation. PLoS One 2011; 6 (12) e28614
- 124 Xu MJ, Cai Y, Wang H. et al. Fat-specific protein 27/CIDEC promotes development of alcoholic steatohepatitis in mice and humans. Gastroenterology 2015; 149 (04) 1030.e6-1041.e6
- 125 Gu Y, Yang Y, Cao X. et al. Plin3 protects against alcoholic liver injury by facilitating lipid export from the endoplasmic reticulum. J Cell Biochem 2019; 120 (09) 16075-16087
- 126 Groebner JL, Girón-Bravo MT, Rothberg ML, Adhikari R, Tuma DJ, Tuma PL. Alcohol-induced microtubule acetylation leads to the accumulation of large, immobile lipid droplets. Am J Physiol Gastrointest Liver Physiol 2019; 317 (04) G373-G386
- 127 Buch S, Stickel F, Trépo E. et al. A genome-wide association study confirms PNPLA3 and identifies TM6SF2 and MBOAT7 as risk loci for alcohol-related cirrhosis. Nat Genet 2015; 47 (12) 1443-1448
- 128 Bianco C, Casirati E, Malvestiti F, Valenti L. Genetic predisposition similarities between NASH and ASH: identification of new therapeutic targets. JHEP Rep Innov Hepatol 2021; 3 (03) 100284
- 129 Li JZ, Huang Y, Karaman R. et al. Chronic overexpression of PNPLA3I148M in mouse liver causes hepatic steatosis. J Clin Invest 2012; 122 (11) 4130-4144
- 130 Teo K, Abeysekera KWM, Adams L. et al; EU-PNAFLD Investigators, GOLD Consortium. rs641738C>T near MBOAT7 is associated with liver fat, ALT and fibrosis in NAFLD: a meta-analysis. J Hepatol 2021; 74 (01) 20-30
- 131 Stickel F, Lutz P, Buch S. et al. Genetic variation in HSD17B13 reduces the risk of developing cirrhosis and hepatocellular carcinoma in alcohol misusers. Hepatology 2020; 72 (01) 88-102
- 132 Mohd Hanafiah K, Groeger J, Flaxman AD, Wiersma ST. Global epidemiology of hepatitis C virus infection: new estimates of age-specific antibody to HCV seroprevalence. Hepatology 2013; 57 (04) 1333-1342
- 133 Ferguson D, Zhang J, Davis MA. et al. The lipid droplet-associated protein perilipin 3 facilitates hepatitis C virus-driven hepatic steatosis. J Lipid Res 2017; 58 (02) 420-432
- 134 [Anonymous]. Global progress report on HIV, viral hepatitis and sexually transmitted infections, 2021. In: Accountability for the global health sector strategies 2016–2021: actions for impact. Geneva: World Health Organization; 2021
- 135 Adinolfi LE, Gambardella M, Andreana A, Tripodi MF, Utili R, Ruggiero G. Steatosis accelerates the progression of liver damage of chronic hepatitis C patients and correlates with specific HCV genotype and visceral obesity. Hepatology 2001; 33 (06) 1358-1364
- 136 André P, Komurian-Pradel F, Deforges S. et al. Characterization of low- and very-low-density hepatitis C virus RNA-containing particles. J Virol 2002; 76 (14) 6919-6928
- 137 Niepmann M. Hepatitis C virus RNA translation. Curr Top Microbiol Immunol 2013; 369: 143-166
- 138 McLauchlan J, Lemberg MK, Hope G, Martoglio B. Intramembrane proteolysis promotes trafficking of hepatitis C virus core protein to lipid droplets. EMBO J 2002; 21 (15) 3980-3988
- 139 Boulant S, Montserret R, Hope RG. et al. Structural determinants that target the hepatitis C virus core protein to lipid droplets. J Biol Chem 2006; 281 (31) 22236-22247
- 140 Pène V, Hernandez C, Vauloup-Fellous C, Garaud-Aunis J, Rosenberg AR. Sequential processing of hepatitis C virus core protein by host cell signal peptidase and signal peptide peptidase: a reassessment. J Viral Hepat 2009; 16 (10) 705-715
- 141 Boulant S, Targett-Adams P, McLauchlan J. Disrupting the association of hepatitis C virus core protein with lipid droplets correlates with a loss in production of infectious virus. J Gen Virol 2007; 88 (Pt 8): 2204-2213
- 142 Miyanari Y, Atsuzawa K, Usuda N. et al. The lipid droplet is an important organelle for hepatitis C virus production. Nat Cell Biol 2007; 9 (09) 1089-1097
- 143 Bartenschlager R, Penin F, Lohmann V, André P. Assembly of infectious hepatitis C virus particles. Trends Microbiol 2011; 19 (02) 95-103
- 144 Sidorkiewicz M. Hepatitis C virus uses host lipids to its own advantage. Metabolites 2021; 11 (05) 11
- 145 Gastaminza P, Cheng G, Wieland S, Zhong J, Liao W, Chisari FV. Cellular determinants of hepatitis C virus assembly, maturation, degradation, and secretion. J Virol 2008; 82 (05) 2120-2129
- 146 Hueging K, Doepke M, Vieyres G. et al. Apolipoprotein E codetermines tissue tropism of hepatitis C virus and is crucial for viral cell-to-cell transmission by contributing to a postenvelopment step of assembly. J Virol 2014; 88 (03) 1433-1446
- 147 Lonardo A, Adinolfi LE, Restivo L. et al. Pathogenesis and significance of hepatitis C virus steatosis: an update on survival strategy of a successful pathogen. World J Gastroenterol 2014; 20 (23) 7089-7103
- 148 Hope RG, McLauchlan J. Sequence motifs required for lipid droplet association and protein stability are unique to the hepatitis C virus core protein. J Gen Virol 2000; 81 (Pt 8): 1913-1925
- 149 Loizides-Mangold U, Clément S, Alfonso-Garcia A. et al. HCV 3a core protein increases lipid droplet cholesteryl ester content via a mechanism dependent on sphingolipid biosynthesis. PLoS One 2014; 9 (12) e115309
- 150 Herker E, Harris C, Hernandez C. et al. Efficient hepatitis C virus particle formation requires diacylglycerol acyltransferase-1. Nat Med 2010; 16 (11) 1295-1298
- 151 Camus G, Herker E, Modi AA. et al. Diacylglycerol acyltransferase-1 localizes hepatitis C virus NS5A protein to lipid droplets and enhances NS5A interaction with the viral capsid core. J Biol Chem 2013; 288 (14) 9915-9923
- 152 Xu N, Zhang SO, Cole RA. et al. The FATP1-DGAT2 complex facilitates lipid droplet expansion at the ER-lipid droplet interface. J Cell Biol 2012; 198 (05) 895-911
- 153 Vieyres G, Pietschmann T. HCV pit stop at the lipid droplet: refuel lipids and put on a lipoprotein coat before exit. Cells 2019; 8 (03) 8
- 154 Lerat H, Kammoun HL, Hainault I. et al. Hepatitis C virus proteins induce lipogenesis and defective triglyceride secretion in transgenic mice. J Biol Chem 2009; 284 (48) 33466-33474
- 155 Lowey B, Hertz L, Chiu S. et al. Hepatitis C virus infection induces hepatic expression of NF-κB-inducing kinase and lipogenesis by downregulating miR-122. mBio 2019; 10 (04) e01617-e01619
- 156 Khatun M, Sur S, Steele R, Ray R, Ray RB. Inhibition of long noncoding RNA Linc-Pint by hepatitis C virus in infected hepatocytes enhances lipogenesis. Hepatology 2021; 74 (01) 41-54
- 157 Vieyres G, Reichert I, Carpentier A, Vondran FWR, Pietschmann T. The ATGL lipase cooperates with ABHD5 to mobilize lipids for hepatitis C virus assembly. PLoS Pathog 2020; 16 (06) e1008554
- 158 Carr RM, Dhir R, Mahadev K. et al. Perilipin staining distinguishes between steatosis and non-alcoholic steatohepatitis in adults and children. Clin Gastroenterol Hepatol 2017; 15 (01) 145-147
- 159 Zhang J, Gao X, Yuan Y. et al. Perilipin 5 alleviates HCV NS5A-induced lipotoxic injuries in liver. Lipids Health Dis 2019; 18 (01) 87
- 160 Salameh H, Masadeh M, Al Hanayneh M. et al. PNPLA3 polymorphism increases risk for and severity of chronic hepatitis C liver disease. World J Hepatol 2016; 8 (35) 1584-1592
- 161 About F, Abel L, Cobat A. HCV-associated liver fibrosis and HSD17B13. N Engl J Med 2018; 379 (19) 1875-1876
- 162 Burlone ME, Bellan M, Barbaglia MN. et al. HSD17B13 and other liver fat-modulating genes predict development of hepatocellular carcinoma among HCV-positive cirrhotics with and without viral clearance after DAA treatment. Clin J Gastroenterol 2022; 15 (02) 301-309
- 163 Listenberger LL, Brown DA. Fluorescent detection of lipid droplets and associated proteins. Curr Protoc Cell Biol 2007 ;Chapter 24:Unit 24.22
- 164 Listenberger LL, Studer AM, Brown DA, Wolins NE. Fluorescent detection of lipid droplets and associated proteins. Curr Protoc Cell Biol 2016; 71: 31.1 , 14
- 165 Qiu B, Simon MC. BODIPY 493/503 staining of neutral lipid droplets for microscopy and quantification by flow cytometry. Bio Protoc 2016; 6 (17) 6
- 166 Chen J, Yue F, Kuang S. Labeling and analyzing lipid droplets in mouse muscle stem cells. STAR Protoc 2022; 3 (04) 101849
- 167 Garcia K, Alves A, Ribeiro-Rodrigues TM, Reis F, Viana S. Analysis of fluorescent-stained lipid droplets with 3d reconstruction for hepatic steatosis assessment. J Vis Exp 2023; (196) e65206 DOI: 10.3791/65206.
- 168 Gojanovich AD, Gimenez MC, Masone D. et al. Human adipose-derived mesenchymal stem/stromal cells handling protocols. lipid droplets and proteins double-staining. Front Cell Dev Biol 2018; 6: 33
- 169 Nishad A, Naseem A, Rani S, Malik S. Automated qualitative batch measurement of lipid droplets in the liver of bird using ImageJ. STAR Protoc 2023; 4 (03) 102466
- 170 Liu CY, Zhu J, Xie Z. Visualizing yeast organelles with fluorescent protein markers. J Vis Exp 2022; (182) e63846 DOI: 10.3791/63846.
- 171 Galli A, Scheel TKH, Prentoe JC, Mikkelsen LS, Gottwein JM, Bukh J. Analysis of hepatitis C virus core/NS5A protein co-localization using novel cell culture systems expressing core-NS2 and NS5A of genotypes 1-7. J Gen Virol 2013; 94 (Pt 10): 2221-2235
- 172 Schüssele DS, Haller PK, Haas ML, Hunter C, Sporbeck K, Proikas-Cezanne T. Autophagy profiling in single cells with open source CellProfiler-based image analysis. Autophagy 2023; 19 (01) 338-351
- 173 Adomshick V, Pu Y, Veiga-Lopez A. Automated lipid droplet quantification system for phenotypic analysis of adipocytes using CellProfiler. Toxicol Mech Methods 2020; 30 (05) 378-387
- 174 Stiebing C, Schmölz L, Wallert M, Matthäus C, Lorkowski S, Popp J. Raman imaging of macrophages incubated with triglyceride-enriched oxLDL visualizes translocation of lipids between endocytic vesicles and lipid droplets. J Lipid Res 2017; 58 (05) 876-883
- 175 Fujimoto T, Ohsaki Y, Suzuki M, Cheng J. Imaging lipid droplets by electron microscopy. Methods Cell Biol 2013; 116: 227-251
- 176 Brasaemle DL, Wolins NE. Isolation of lipid droplets from cells by density gradient centrifugation. Curr Protoc Cell Biol 2016; 72: 3.15.1-3.15.13
- 177 Ding Y, Zhang S, Yang L. et al. Isolating lipid droplets from multiple species. Nat Protoc 2013; 8 (01) 43-51
- 178 Mannik J, Meyers A, Dalhaimer P. Isolation of cellular lipid droplets: two purification techniques starting from yeast cells and human placentas. J Vis Exp 2014; (86) 50981 DOI: 10.3791/50981.
- 179 Brasaemle DL, Wolins NE. Isolation of lipid droplets from cells by density gradient centrifugation. Curr Protoc Cell Biol 2016; 72: 15.1 , 13
- 180 Rösch K, Kwiatkowski M, Schlüter H, Herker E. Lipid droplet isolation for quantitative mass spectrometry analysis. J Vis Exp 2017; (122) 55585
- 181 Brettschneider J, Correnti JM, Lin C, Williams B, Oranu A, Kuriakose A, McIver-Jenkins D, Haba A, Kaneza I, Jeon S, Scorletti E, Carr RM. Rapid lipid droplet isolation protocol using a well-established organelle isolation kit. J Vis Exp 2019; (146) e59290 DOI: 10.3791/59290.
- 182 Kamerkar S, Singh J, Tripathy S, Bhonsle H, Kumar M, Mallik R. Metabolic and immune-sensitive contacts between lipid droplets and endoplasmic reticulum reconstituted in vitro. Proc Natl Acad Sci U S A 2022; 119 (24) e2200513119
- 183 Preuss C, Jelenik T, Bódis K. et al. A new targeted lipidomics approach reveals lipid droplets in liver, muscle and heart as a repository for diacylglycerol and ceramide species in non-alcoholic fatty liver. Cells 2019; 8 (03) 8
- 184 Rémy C, Fouilhé N, Barba I. et al. Evidence that mobile lipids detected in rat brain glioma by 1H nuclear magnetic resonance correspond to lipid droplets. Cancer Res 1997; 57 (03) 407-414
- 185 Bartz R, Li WH, Venables B. et al. Lipidomics reveals that adiposomes store ether lipids and mediate phospholipid traffic. J Lipid Res 2007; 48 (04) 837-847
- 186 Nigam S, Ranjan R, Sinha N, Ateeq B. Nuclear magnetic resonance spectroscopy reveals dysregulation of monounsaturated fatty acid metabolism upon SPINK1 attenuation in colorectal cancer. NMR Biomed 2022; 35 (07) e4705
- 187 Schmidt C, Ploier B, Koch B, Daum G. Analysis of yeast lipid droplet proteome and lipidome. Methods Cell Biol 2013; 116: 15-37
- 188 Lagrutta LC, Layerenza JP, Bronsoms S, Trejo SA, Ves-Losada A. Nuclear-lipid-droplet proteome: carboxylesterase as a nuclear lipase involved in lipid-droplet homeostasis. Heliyon 2021; 7 (03) e06539
- 189 Peterson CWH, Deol KK, To M, Olzmann JA. Optimized protocol for the identification of lipid droplet proteomes using proximity labeling proteomics in cultured human cells. STAR Protoc 2021; 2 (02) 100579
- 190 Tatenaka Y, Kato H, Ishiyama M. et al. Monitoring lipid droplet dynamics in living cells by using fluorescent probes. Biochemistry 2019; 58 (06) 499-503
- 191 Ma X, Zhi Z, Zhang S, Liu P. Measurement of ATGL activity using adiposomes. Biophys Rep 2023; 9 (01) 3-14
- 192 Bridge-Comer PE, Reilly SM. Measuring the rate of lipolysis in ex vivo murine adipose tissue and primary preadipocytes differentiated in vitro. J Vis Exp 2023; (193) e65106 DOI: 10.3791/65106.
- 193 Chorlay A, Santinho A, Thiam AR. Making droplet-embedded vesicles to model cellular lipid droplets. STAR Protoc 2020; 1 (03) 100116
- 194 Zhi Z, Ma X, Zhou C, Mechler A, Zhang S, Liu P. Protocol for using artificial lipid droplets to study the binding affinity of lipid droplet-associated proteins. STAR Protoc 2022; 3 (01) 101214
- 195 Drew L. Drug development: sprint finish. Nature 2017; 551 (7681) 551
- 196 A study to assess safety, tolerability, PK and PD of AZD2693 in non-alcoholic steatohepatitis patients. Accessed September 25, 2023, at: https://clinicaltrials.gov/ct2/show/NCT04483947
- 197 Lindén D, Ahnmark A, Pingitore P. et al. Pnpla3 silencing with antisense oligonucleotides ameliorates nonalcoholic steatohepatitis and fibrosis in Pnpla3 I148M knock-in mice. Mol Metab 2019; 22: 49-61
- 198 Knockdown of HSD17B13 mRNA, pharmacokinetics, safety, and tolerability, of AZD7503 in non-alcoholic fatty liver disease. Accessed September 25, 2023, at: https://clinicaltrials.gov/ct2/show/NCT05560607




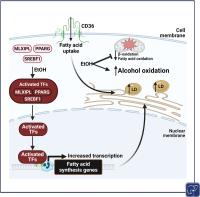


